*Check the website program below for the latest updates
Daily Schedule at a Glance
Fleming-T1
The fundamentals of ion migration in crystalline ionic solids are well established. Ion migration is essentially a classical process, owing to the high masses of the ions. It is mediated by point defects, either vacancies or interstitials. It is thermally activated, exhibiting Arrhenius behaviour, with an activation enthalpy of defect migration that varies, according to the ion that is moving and the crystal structure in which the ion is moving, from a fraction of an eV to several eV.
Determining experimentally for a specific material the point defect that is responsible for ion migration and its associated migration enthalpy are challenging tasks. Computer simulations have proved extremely helpful in this regard, by providing data for closely controlled systems, by aiding the interpretation of experimental data and by providing atomic-scale insights. Caution needs to be exercised, however, in performing simulations and in using them to interpret experimental data. In this presentation, focussing on ion migration in crystalline solids, I will examine various themes from this intersection of experiment and simulation for which caution is required.
Fleming-K1
2005 Dr. rer. nat. in Physical Chemistry, University of Hannover (Germany) advisor P. Heitjans
2011 Habilitation (Dr. habil.) in Physical Chemistry, University of Hannover (Germany)
2011 Full Professor at the TU Graz (Austria)
2012 Director of the Christian Doppler lab 'Materials for Lithium Batteries'
2016 Director of the Institute (ICTM) at the TU Graz
2023 Vice Dean of the Faculty TCVB / Prodekan
Nuclear magnetic resonance offers plenty of advanced techniques, such as 1D and 2D EXSY [1,2], to study dynamic features of solid-state lithium ion conductors. Provided ultrafast ion dynamics is present at temperatures around ambient, i.e., dealing with jump rates in the order of some GHz, extremely low, if not cryogenic, temperatures are needed to completely freeze any Li+ ion dynamics on the NMR time scale. Taking advantage of two substances, viz. Ge-bearing Li6PS5I [3] and LiTi2(PS4)3 [4], we measured 7Li NMR line shapes and spin-lattice relaxation rates over a wide temperature range down to 9 K. The NMR experiments resolve the various Li+ exchange processes that ultimately give rise to overall, fast ionic transport at ambient conditions. As an example, for Ge-containing Li6PS5I we identified the spin-lock NMR rate peak appearing at 163 K as the one reflecting the rate-limiting intercage Li+ diffusion process that enables the ions to be transported over long distances. The corresponding Einstein-Smoluchowski diffusion coefficient excellently agrees with that indirectly probed by macroscopic conductivity spectroscopy.
Fleming-O1
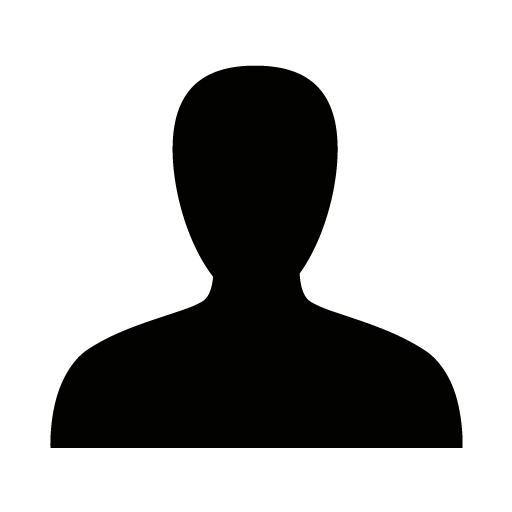
All Solid-State Batteries (ASSBs) are looked as the most promising technology to go beyond the limitation of LiBs. The presence of a solid electrolyte may reduce the propagation of dendrites, and absence of a flammable liquid eases the explosion risk in case of thermal runaway. This kind of batteries, denominated Gen4b, couples the solid electrolyte with Li metal (theoretical specific capacity: 3860 mA h/g) and high voltage cathodes, which use, allowed by the low flammable risk, will in addition increase the gravimetric and volumetric energy density beyond incumbent LIBs[1].
Sulfide-based materials emerge from the historical proposed ceramic materials, such oxides, as a favorable candidate to be used as solid electrolyte in ASSBs in view of their promising performance and ease of manufacturing. Sulfides are characterized by high ionic conductivity (above 1mS/cm at RT), they show mechanical strength together with easy processability, which allows their manufacturing from densification under pressure at room temperature (an advantage towards oxides, which require high sintering temperature). However, their difficulty to withstand high current density and long-term cycling, as well as their sensitivity to moisture are hampering their entrance into the battery market.
This work aims to provide a better understanding of the transport properties of sulfide-based ASSBs, for which, as a case of study, argyrodite Li6PS5Cl separators are considered. First the conduction properties were analyzed and correlated with Li-ion mobility in the electrolyte itself. Secondly the interfacial processes taking place at the battery level were investigated, for this purpose, distribution of relaxation times has been applied[2,3]. This technique allows to correlate the factors determining the cell performance to the various impedance sources happening at the Li|electrolyte and cathode|electrolyte interfaces. As result, the degradation process at the electrolyte interfaces have been followed during cycling, giving precious insight on the parasitic reaction happening during real operating condition.
Westminster-K1
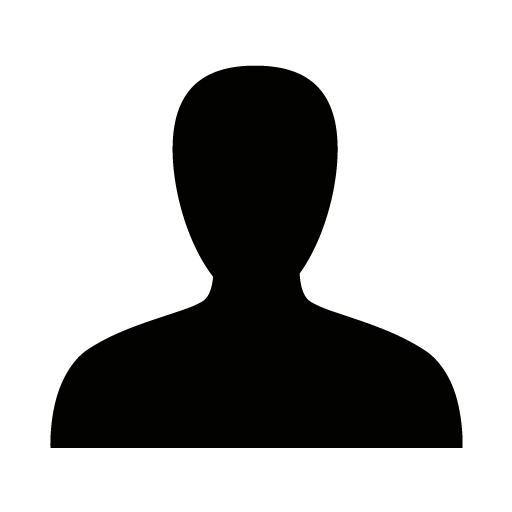
Conductivity degradation is one of the key limitations in the commercial implementation of doped zirconia electrolytes. This closely aligns with odering phenomena in both the anion and cation lattices. We briefly review these phenomena and illustrate this with a special example, a magnesia and india co-doped zirconia composition that exhibits an unusual conductivity degradation-recovery profile where the conductivity degrades for a short period of time then recovers and exceeds its initial value. The conductivity at 850 °C in air was found recovered to the initial value of 0.096 S cm-1 in less than 25 hours, and then it continues to improve up to 0. 11 S cm-1 after 250 hours. This special behaviour in a stable single-phased composition allows us to unambiguously correlate conductivity changes over time to changes in short-range structure. Through a detailed structural characterisation by electron diffraction, TOF neutron diffraction and other techniques, the long-standing recognition that the conductivity degradation is related to short-range ordering is confirmed experimentally. We conclude that the conductivity degradation is caused by pyrochlore and rare-earth C-type ordering within the fluorite, whereas the conductivity increase and stabilisation observed in this composition is a result of eliminating such defect short-range ordering and moving towards a stable disordered fluorite structures.
Westminster-O1
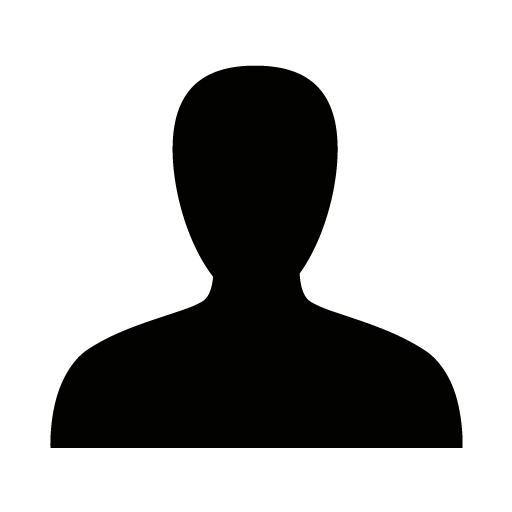
Ceria and its solid solutions exhibit excellent redox properties and stability in a wide range of temperatures and oxygen partial pressures (pO2). These materials therefore find applications in solid oxide cells (SOCs), oxygen separation membranes, catalytic surface coatings, and oxygen sensors. In the present study, we have investigated the electrical conductivity of Gd0.10Ce0.90O2-δ (GCO) as a function of both pO2 (10-0.36-10-25 bar) and temperature (650-850 ͦC). Experimental data are modeled in terms of the underlying defect chemistry. Our work clearly highlights departures from ideal solution behavior by concurrently modeling data of oxygen non-stoichiometry and electrical conductivity. Our analysis starts with analyzing literature data on the oxygen non-stoichiometry of GCO.1 Following Mizusaki et al.2,3, and in agreement with earlier reports for doped ceria,4,5 we demonstrate that the observed decrease in reduction enthalpy for GCO with decreasing pO2 can be accounted for by the interactions between the involved ionic and electronic defects. The non-ideality of the experimental data of electrical conductivity can also be traced back to the interactions of defects. The results indicate that upon reduction the small polarons formed in GCO retain their mobile character, rather than being trapped in complex defect associates as has been suggested previously.1
St.James-K1
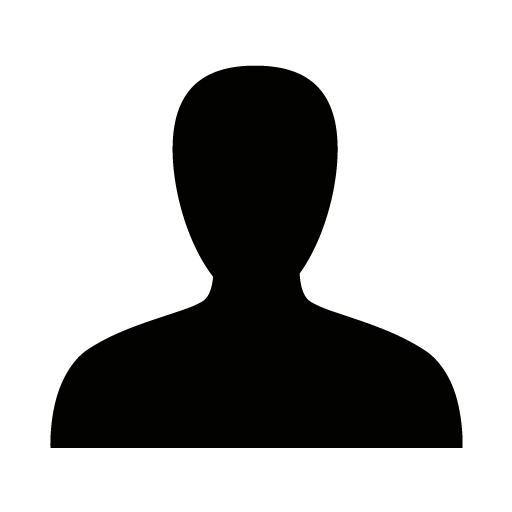
Over the past four decades, protonic ceramics have captivated increasing attention, with a notable surge in interest observed in recent years. This trend can be explained by a better understanding of their properties and the development of scalable and reproducible manufacturing processes.
This presentation will begin with a comprehensive overview of protonic ceramics, specifically emphasizing their conductivity and hydration properties. Subsequently, it will delve into the diverse applications of protonic ceramic cells, encompassing fuel cells, electrolyzers, and membrane reactors. The core of the presentation will revolve around electrolysis cells (protonic ceramic electrolysis cells, PCECs), with an initial focus on the latest advancements in the field. Following this, the major challenges and corresponding solutions will be addressed:
(1) The faradaic efficiency, which is proportional to the ratio of the net hydrogen molar flux over the imposed current density, is an important performance metric: the discrepancy in the results in the literature and potential solutions to achieve high faradaic efficiencies will be discussed [1];
(2) The steam electrode (positrode) mechanisms are more complex than in solid oxide cells, and the community is working on developing highly performant materials at intermediate temperatures [2]. In addition, the positrode materials must also be stable under high steam contents.
St.James-O1
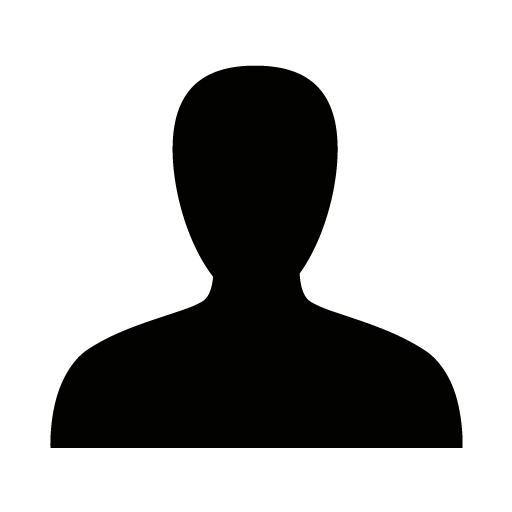
Proton-conducting oxides are extremely promising for playing a central role in future energy supplies based on renewables – for example as electrolyte and electrode materials in solid oxide fuel or electrolysis cells, or as mixed proton- and electron-conducting ceramic membranes for hydrogen purification. Materials with perovskite-type structures have emerged as possible candidates for both applications. However, their composition, and in particular, their basicity influence the achievable concentration of protons and thus the ionic conductivity of the material. What makes the situation even more difficult is that the proton concentration in an oxide cannot be easily measured, especially not in-situ, which would be important if one wants to obtain this information on a running device.
This study is dedicated to examining the achievable proton concentration in the perovskite-type model material BaFe0.8Y0.2O3-δ (BFY) in various oxidation and protonation states, as the protonation of BFY is intricately linked to the oxidation state of iron. Since this material is an auspicious candidate as a potential electrode material in proton-conducting fuel/electrolysis cells, its proton uptake has already been extensively studied, revealing favorable behavior[1]. The determination of the proton concentration in BFY, however, remains a challenge and primarily relies on techniques that involve indirect analysis of protons, such as thermogravimetry and conductivity measurements, which are considered state-of-the-art methods.
Here we present two alternative analytical methods that can be used for direct detection of protons in perovskites and are also very well suited for in-situ quantification: Infrared (IR) spectroscopy and laser induced breakdown spectroscopy (LIBS). IR spectroscopy provides a potential avenue for directly analyzing protons, since they can be expected to exhibit a characteristic OH vibration band. Indeed such an absorption band is observable on BFY, and its position in the IR spectrum is in good accordance with simulations in literature[2]. LIBS allows direct measurement of the proton concentration via analyzing the characteristic emission from the plasma generated by irradiating the material with an UV-laser. Here we present a customized sample chamber that enables LIBS measurements in different and even changing atmospheres, allowing in-situ analysis of protons in oxides.
The results obtained were validated by gravimetric measurements and supplemented by X-ray diffraction analyses, and both techniques demonstrated their ability to directly detect protons in perovskite-type oxides. This is particularly noteworthy as it makes them immune to artifacts arising from competing reactions, such as oxygen uptake, a challenge often encountered in thermogravimetry. The findings underscore the reliability of our analytical approach, and validate it as a valuable alternative to conventional methods, thus contributing to advancements in renewable energy applications.
Moore-K1
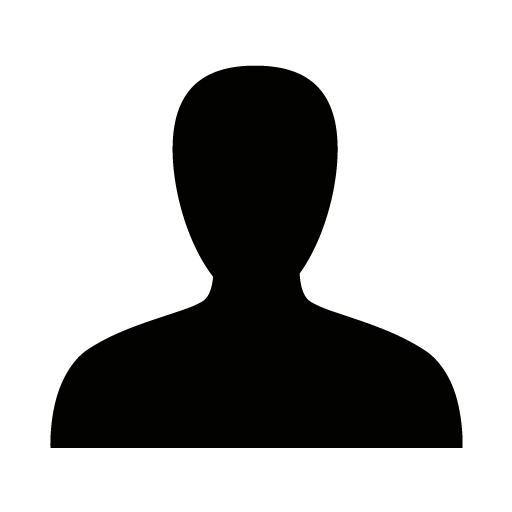
The fundamental relationships between performance metrics, impedance spectra, and electrochemical processes in electrolysis devices and other electrochemical systems are highly complex and often difficult to disentangle. This lack of knowledge hinders identification and evaluation of physicochemical processes, limits understanding of how materials and device architecture influence performance, and ultimately inhibits principled design choices. There is a need for practical, robust procedures to gain physical insight into electrochemical device performance. This requires effective design of experiments to sufficiently characterize varying conditions within reasonable time constraints. In addition, new methods for coherent analysis of large electrochemical datasets are necessary to extract meaning from vast amounts of raw data.
Here, we introduce a novel approach to accelerate electrochemical characterization with standard instrumentation by utilizing rapid measurements in both the time and frequency domains. This “hybrid electrochemical impedance spectroscopy” (HEIS) method provides excellent resolution across a broad range of timescales while decreasing measurement time by more than an order of magnitude compared to conventional electrochemical impedance spectroscopy. The technique can be applied to quickly construct detailed electrochemical maps of energy conversion devices across multiple measurement condition dimensions, revealing physicochemical relationships that are hidden in sparse conventional datasets. We use this new approach to comprehensively characterize a commercial Li-ion battery as a function of C-rate and state-of-charge during charging/discharging and a reversible protonic ceramic electrolyzer/fuel cell (PCEC) under different temperature, atmosphere, and bias conditions. Altogether, more than 20,000 distinct HEIS experiments are performed, resulting in a rich dataset that can be assembled to form relaxation hypersurfaces - multi-dimensional analogs of the one-dimensional distribution of relaxation times (DRT) - which are then processed with new analysis techniques to reveal the underlying processes that govern device performance. This approach describes the electrochemical behavior of both batteries and PCECs with an unprecedented level of detail made possible by accelerated measurement and scalable data processing strategies. However, this study represents just one possible form of the mapping concept. The underlying principles and techniques can be adapted to develop various new ways to examine a wide variety of energy-conversion devices. The conceptual approach demonstrated here has the potential to reduce the time required to understand electrochemical materials and device architectures, enabling a faster design cycle.
Moore-O1
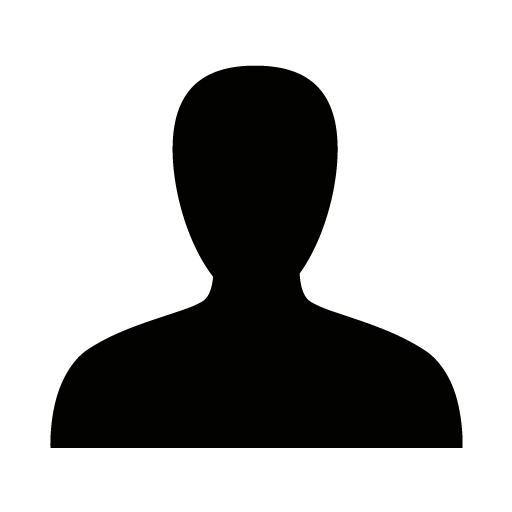
Electrochemical impedance spectroscopy is a powerful tool to monitor systems in operation. The analysis of impedance data mostly involves pattern recognition and fitting with a simple electric equivalent circuit model. This has become the standard for studying liquid systems, but the analysis of inhomogeneous solid-state systems is more complex. This is due to the microstructure of the sample and the morphology of interfaces, which introduce additional degrees of freedom. As a result, the transport through real structures cannot be adequately described by 1D models. However, this is typically neglected when analyzing the impedance in terms of the transport processes taking place in the system, which in turn can lead to serious misinterpretations.
For example, diffusion processes on the metal anode interface strongly influence the cycling performance of a solid-state battery. This includes charge transfer driven morphological (in)stabilities, e.g., at the interface between lithium and Li6.25Al0.25La3Zr2O12 (LLZO). For a long time, the interface impedance response has been mistakenly attributed to charge transfer reactions, leading to the misconception that an inherently high transfer resistance prevents the realization of the reversible metal anode concept. However, experiments by Krauskopf et al. have shown that the actual cause is the non-ideal physical contact between lithium and LLZO, i.e., the presence of pores at the interface.[1] The charge transfer resistance, on the other hand, is negligible.
Although the current constriction effect is well-known as a DC phenomenon, its AC behavior is not yet fully understood. Thus, we have built on earlier work by Fleig and Maier[2],[3] to understand the various dependencies of the constriction phenomenon, e.g., on the microstructure of LLZO, or the interface morphology. We show that the signature of the current constriction signal in the impedance spectrum is identical to that of ion transport. However, it is not a migration process in the strict sense. It is related to the frequency-dependent change of the electrode area that actively contributes to the transport.[4],[5] It is a geometric effect that is expected at different length scales.
As a result, various processes (e.g., charge transfer reactions, morphological and chemical instabilities, etc.) affect the interface properties of metal anodes. Identifying the dominant interface effect(s) is crucial, as the strategy for improving a solid-state battery depends on the rate-limiting process that needs to be overcome. For this purpose, we have developed a guideline for the interpretation of experimental impedance data of parent metal anodes to increase the reliability of future impedance analyses.[6]
Abbey-K1
The advent of solid-state batteries has spawned a recent increase in interest in lithium conducting solid electrolytes. However, many open questions remain when trying to optimize electrolytes and understand solid state battery chemistries.
In this presentation, we will show that it is not only important to find fast ionic conductors, but that fast ionic conduction is paramount within solid state battery composites. Measuring the effective ionic transport in electrode composites provides an avenue to explore transport and stability limitations that in turn provide better criteria for solid state battery performance. These transport limitations will be explored as a function of materials composition, particle sizes as well as temperatures.
By understanding these limitations, we will then explore two approaches to overcome these transport limitations.
Finally, we will show how pressure induces dislocation densities in solid ionic conductors. By increasing the strain and dislocations, the overall ionic transport properties improve extending to solid state battery performance.
Abbey-O1
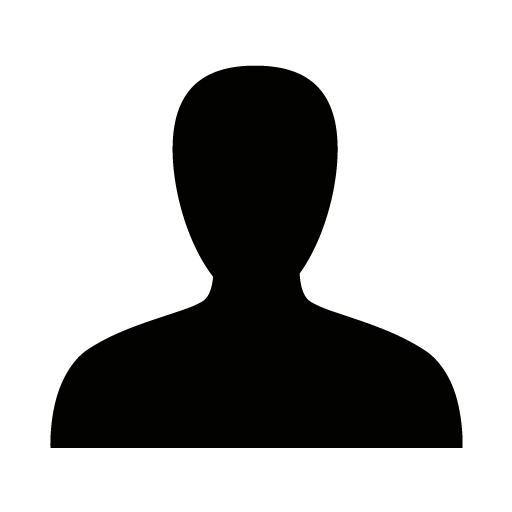
Li(FSA)(SN)2 molecular crystal solid electrolytes, consisting of lithium bis-(fluorosulfonyl)imide (LiFSA) and succinonitrile (SN), exhibit comparable ionic conductivity (1.1×10-4 S·cm-1) and improved transference number (0.95) with traditional solid polymer electrolytes [1]. FSA anions in an ionic liquid electrolyte contribute to the passivation layer formed on the positive electrode surface [2], suggesting that Li(FSA)(SN)2 may enable battery operation under high voltage by passivating the interface. However, the adaptability of Li(FSA)(SN)2 solid electrolytes in high-voltage operation is still unclear. In this study, we fabricated a battery using a thin-film 5 V-class LiNi0.5Mn1.5O4 (LNMO) positive electrode and investigated the operation of the battery under high voltages.
In the early cycles, the battery composed of Li(FSA)(SN)2 and LNMO shows successful charge and discharge cycles up to 4.8 V vs. Li/Li+. However, the capacity shows severe degradation (54% after 100 cycles with the current density of 10 μA·cm-2). Continuous interfacial side reactions and thick interphase layer formation at the Li(FSA)(SN)2 | LNMO interface may have led to capacity degradation. An insufficient passivation of the interface is suggested. Therefore, a highly stable amorphous Li3PO4 buffer layer was inserted on the Li(FSA)(SN)2 | LNMO interface to passivate the interface. With the insertion of Li3PO4, the capacity degradation was suppressed to 4% after 100 charge-discharge cycles, indicating that the insertion of Li3PO4 stabilized the interface even at a high voltage of 4.8 V vs. Li/Li+. This study reveals the potential of molecular crystal solid electrolytes for 5 V-class all-solid-state batteries and highlights the importance of interfacial engineering on battery performance.
Gielgud-K1
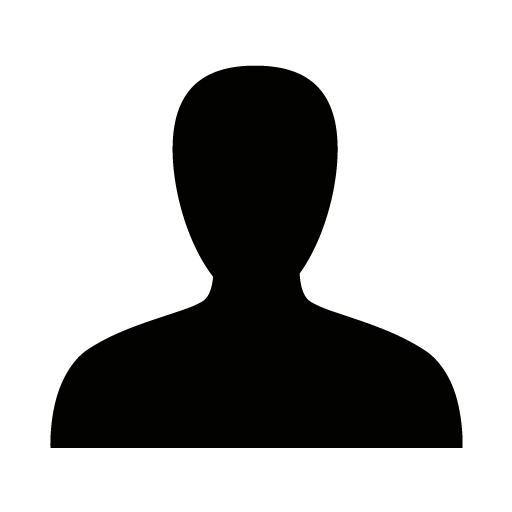
SOEC has a huge potential as an enabling technology to facilitate the transition of the global energy system to a sustainable one. The technology offers unrivalled efficiency in hydrogen generation and has a potential to reach low cost. Generation of hydrogen is the first step in realizing a range of P2X-schemes for providing green fuels (e.g. methanol, ammonia or jet fuel) for the transport sector [1,2].
The largest SOEC units manufactured/operated today are in the range of ~1 MW. However, numerous scale up projects are under way and industrial stakeholders are these years investing massively to bring up production capacity.
In this paper we shall discuss some of the challenges in upscaling SOECs and address some materials issues/opportunities for improving SOEC performance with view to large scale deployment.
The brittle nature of the ceramics used in the cells limits the size of the cells to edge lengths in the range of 10-15 cm. Ideally this should be larger, however, this requires care in the manufacturing, and a tough and strong backbone in the cell. Many stakeholders rely on zirconia to provide the structural backbone of the cell. Transformable metastable tetragonal with 2 – 3.5 mol.% Y2O3 substitution is advantageous over fully stabilized cubic version with ~8 mol. % Y2O3, as the former has higher strength and toughness. We shall present recent results on optimizing composition and processing routes to maximize toughness and strength, whilst preventing unintended spontaneous transformation to the monoclinic phase during use.
In SOEC one does not need any PGM-materials. However, typically the state of the art cells relies on use of several critical raw materials. Several LREE/HREE materials are used (Ce, Gd, Y, La) and also some critical transition metals like Co/Mn and Ni. For large-scale deployment, it is obviously an advantage if the use of these Critical Raw Materials can be minimized. Introducing performance improvements in terms of production capacity per m2 of cell or kg of material is an important strategy to reduce the CRM-use and is likely a part of the strategy among most stakeholders.
We shall here present recent results on activities to increase the competitiveness of the SOEC technology by introducing a metal-support as the structural backbone and replace the Ni/YSZ-fuel electrode with one based on a mixed conducting Ti-based oxide (Ni and Fe-doped (La,Sr)TiO3). Recently, we have found that such a material combination can sustain SOEC operation for extended periods (tested over 5000 hr) at current densities exceeding 0.5 A/cm2. These durability results shall be presented as shall be findings on the fundamental charge transport properties of the titanates.
Finally, results of endeavors to improve electrode performance of both fuel and oxygen electrodes will be discussed. On the fuel electrode side, opportunities and challenges of using Ni/CGO in thin electrolyte cells will be addressed and on the oxygen electrode side, results on boosting of performance via various infiltrates will be summarized.
Gielgud-O1
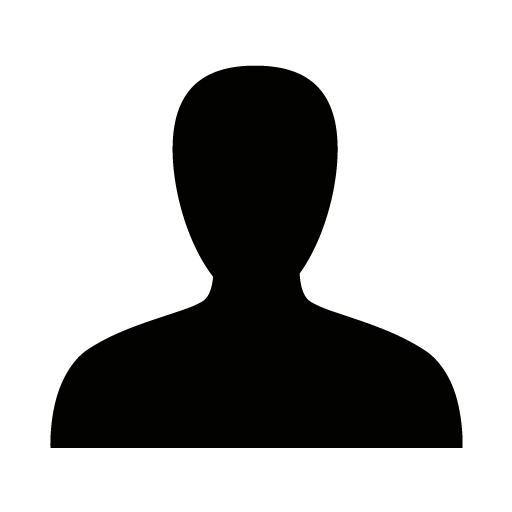
Nowadays, climate changes attributed to the continuous and excessive consumption of carbon-based fossil fuels are causing escalating concerns, leading to a growing demand for sustainable energy technologies. However, the intermittent nature of many renewable energy sources, such as wind and solar, necessitates the development of reliable energy storage technologies. Among the most promising technologies, solid oxide electrolysis cells (SOECs) can convert electrical energy into chemical energy at high operating temperatures (typically 500-1000 ℃), offering the advantage of achieving higher efficiency and lower capital expenditures [1]. Despite their potential, SOECs are not without challenges. The performance of state-of-the-art cells tends to degrade over time, with a significant portion of this degradation originating from the Ni/ytttria-stabilized zirconia (YSZ) electrode [2]. This study aims to investigate the influence on electrochemical performance and microstructure of Ni/YSZ fuel electrode based SOEC, by adding two common impurities, namely Si and Al. As refractory compounds, silica and alumina are frequently used in SOEC systems at both laboratory and industry scales, serving various roles including glass sealing, insulation, and as parts in vacuum pumps and heating elements to mention a few [3,4]. Alumina is also used as a sintering aid in SOEC cells to reduce the sintering temperature [5]. In this study, a Ni contact layer containing alumina and silica was applied on the top of fuel electrode, to mimic accelerated degradation caused by Si/Al impurities. The electrochemical performance and microstructure information were investigated in detail and compared with a cell without such contaminated layer. Based on the results, possible explanation on the mechanism of Si/Al poisoning the Ni/YSZ SOEC cathode was provided.
Fleming-I1
Thin layers have traditionally represented exceptional model systems for fundamental prospects in materials science. However, the extent of such an approach to be utilized in real devices e.g., for energy conversion and storage, is still limited. To date, one of the few successful examples of a commercial thin film energy device is the lithium solid-state battery (SSB) based on a lithium phosphorus oxynitride (LiPON) electrolyte. LiPON remains as the only option in this arena thanks to its stability towards Li-metal and low electronic conductivity. However, LiPON’s low ionic conductivity (c.a. 1 μS·cm-1) limits cells’ performance and its difficult processability hinders large-scale production. Among the alternative ceramic electrolytes, NASICON superionic solid electrolyte Li1+xAlxTi2-x(PO4)3 (LATP) is very promising due to its good ionic conductivity (c.a. 1 mS·cm-1) and stability in ambient air at high temperatures. Despite these advantages, all-ceramic devices based on LATP have not been reported, mainly due to interfacial reactivity between components.
In the present talk, we will present our latest advances in the implementation of thin films in ceramic SSBs and the development of novel investigation tools for materials research and device integration. With this aim, LATP and different electrode materials were deposited by Large-Area Pulsed Laser Deposition. Spinel LiMn2O4 and Li4Ti5O12, and olivine LiFePO4 were chosen, aiming to exploit their outstanding stability, low cost, and environmental friendliness. Bi-layers of LATP with these electrodes were fabricated in order to study the structure of the different interfaces. Various strategies such as the introduction of protective coatings or Rapid Thermal Processing (RTP) procedures are implemented with the aim of improving the quality of these electrode-electrolyte interfaces. The nature of such interfaces and their evolution after being subjected to electrochemical cycles has been examined from different perspectives applying a series of complementary techniques such as Focused Ion Beam-Secondary Ion Mass Spectroscopy (FIB-SIMS), Atom Probe Tomography (APT), Grazing Incidence Wide Angle X-Ray Scattering (GIWAXS), among others. Our results show the interface chemical stability and electrochemical performance of these bi-layers demonstrating their suitability for future thin-film solid state lithium batteries (SSLBs).
Fleming-O1
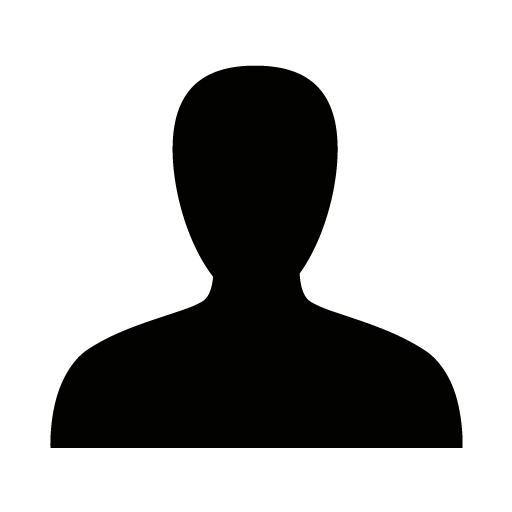
All-solid-state lithium-ion batteries are expected as one of the energy storage technologies of next generation. In contrast to sulfide-based solid electrolytes, which can be densified via simple pressing to achieve sufficient ionic conductance, application of oxide-based solid electrolytes generally requires high-temperature sintering for its densification. Considering cell fabrication via co-sintering of electrolyte and active material, high process temperature may lead to undesirable reaction between them and thereby degraded interfacial conductance. Thus low-temperature sintering of oxide electrolytes is an important processing technology.
In the preceding study on co-firing process for all-solid-state battery, it was found that a NASICON-type lithium-ion conducting electrolyte Li1.3Al0.3Ti1.7(PO4)3 (LATP) in contact with LiCoPO4 shows quite high sinterability. In order to understand the mechanism of the sinterability enhancement, the present authors have investigated the influence of cobalt-introduction on the sintering behavior of LATP as well as the phase equilibrium. It was suggested that 1~2 mol% of cobalt is allowed to substitute for the constituent cation in the NASICON structure, leaving no traces of Co-including second phases in the XRD patterns. The slightly Co-doped LATP phase shows a moderately high sinterability; the relative density approaches 80% after firing at 800°C, while a normal LATP undergoes almost no densification at the same temperature. Further increase in the cobalt content results in the excess of cobalt in reference to the solubility limit in the LATP phase, forming LiCoPO4 together with the other second phases. In the case of the nominal composition such that titanium in LATP is partly replaced by cobalt (Li1.3+2xAl0.3CoxTi1.7-x(PO4)3), a significant densification was observed; e.g., a relative density approximating 100% was attained after firing at 800°C for x=0.1. The significantly high sinterability is ascribed to a low melting-point compound LiPO3, which forms as the second phase together with LiCoPO4 and LiTiO(PO4).
Fleming-O2
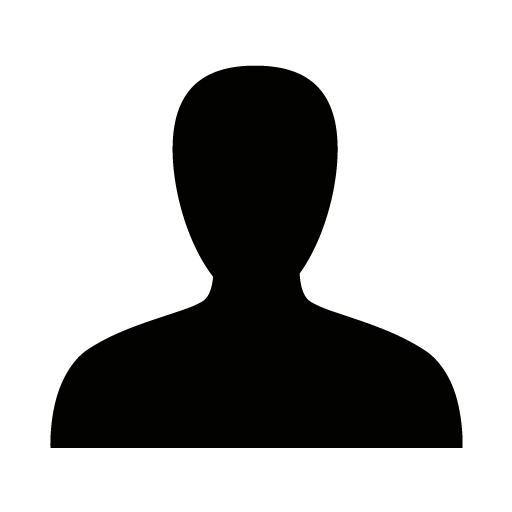
All-solid-state lithium-ion batteries is considered a front runner among next-generation battery technologies. Solid-electrolytes which allows for Li-ion only mobility and practically inflammable nature, it solves safety and cross migration issues. Additionally, all solid-sate batteries promise significantly high energy as well as power densities. [1], [2] Among the many challenges pertaining to all-solid-state batteries, the electrochemical stability of solid-electrolytes as well as the interfaces remains a key challenge. Recent computational as well as experimental studies have shown that many of these lithium-ion solid electrolytes has poor electrochemical stability at high voltage. [3]–[5] Lithium-stuffed garnet-type solid-electrolyte is one the most widely studied material for all-solid-state batteries for its’ high ionic conductivity and kinetic stability against lithium metal anode. [6], [7] The computational works for Li7La3Zr2O12 (LLZO) garnets have predicted poor electrochemical stability, with oxidation onsetting as low as 2.9 V vs. Li/Li+. [8], [9] Indeed, poor cycling performance has been noted in many experimental studies employing LLZO-type garnets solid-electrolytes, [10]–[12] which among other factors, possibly, implicate as predicted low oxidation potential. Earlier studies based on utilizing electrochemical techniques have suggested oxidative decomposition at potential as low as 3.7 V vs. Li/Li+. [9], [13], [14]. Nonetheless, there remains a gap in the literature for local structural changes in these solid-electrolytes in response to relevant high electrochemical potential.
In this study, we studied local structural and impedance change of representative Al-doped LLZO garnet solid electrolyte as a result of electrochemical reactivity. The experimental techniques utilized the Zr K-edge extended x-ray absorption fine structure (EXAFS) to characterize the local structure and O K-edge soft x-ray absorption spectroscopy (XAS) to deconvolute degradation products. The EXAFS spectra were collected at Advanced Photon Source (APS) beamline 10-ID-B, Argonne National Laboratory. The O K-edge XAS was collected at beamline 23-ID-2 at NSLS-II, Brookhaven National Laboratory.The results shows that Zr coordination shells in LLZO underwent major shrinkage with added, seemingly, loss of coordination number. These results are in agreement with electrochemical induced loss of oxygen from the lattice, a mechanism reported earlier. [9], [15] The O K-edge XAS which further confirms the degradation of LLZO by showing suppression of its’ signature feature as a result of electrochemical oxidation. The growth of impedance after polarization suggest Li-mobility is significantly reduced as a result of electrochemical reactivity at higher potential. In summary, our results show significant local structural change in garnet-type solid-electrolytes under electrochemical potentials at 4.3 V vs. Li/Li+. These local changes in structure directly impedes Li-ion mobility. Cell degradation at these potentials could result from oxidation instability of the garnet electrolyte.
Fleming-O3
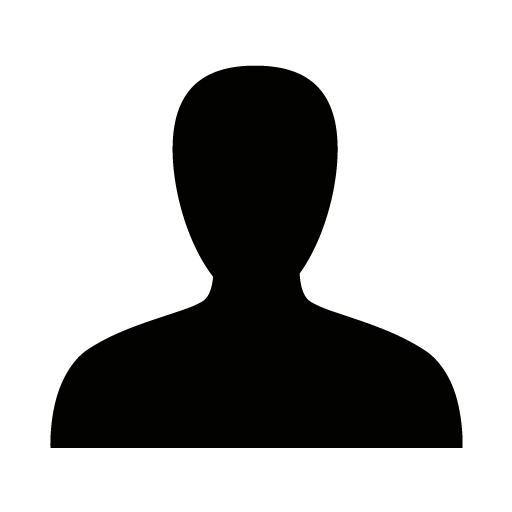
With the rapidly growing EV industry, the development of safer batteries with solid-state electrolytes is highly important. The design of composite polymer electrolytes (CPE) incorporating Li+ conductor ceramic particles inside a polymeric matrix is an interesting approach to combine the flexibility and processability of the polymers with the high ionic conductivity and electrochemical stability of ceramics. Despite the improved stability of these electrolytes, their ionic conductivity is insufficient for high-rate applications. To address this issue, a heat treatment process was proposed to remove the secondary phases from the surface of the ceramic particles and form a better interphase with the polymer matrix.
In this work, solid-state NMR spectroscopy was applied to investigate the chemical and phase evolution of LLZO garnets during heat treatment and its impact on the local Li dynamics. Using relaxometry, 1H-7Li heteronuclear correlations, and variable temperature NMR experiments, it was shown that heat treatment can effectively remove the LiOH and Li2CO3 from the surface of LLZO, and eliminate the protons from its bulk, leading to a significant improvement in the Li dynamics in LLZO powders.[1] Careful analysis of the 7Li spectra showed that removing the secondary phases on the surface of LLZO also improves the local interactions between the PEO and LLZO, ultimately leading to higher ionic conductivity and mechanical properties.[2] In the next step, 2D 7Li-7Li and 6Li-6Li EXSY NMR experiments were employed to detect the Li-ion exchange and quantify the exchange rates. Using pseudo-3D experiments, LiOH was identified as an intermediate phase for the interfacial Li exchange. Finally, 7Li → 6Li trace exchange experiments were employed to determine the Li-ion pathway through the electrolyte. It was concluded that the heat treatment of LLZO is essential for achieving CPEs with improved micro and macro-scale properties.
Westminster-I1
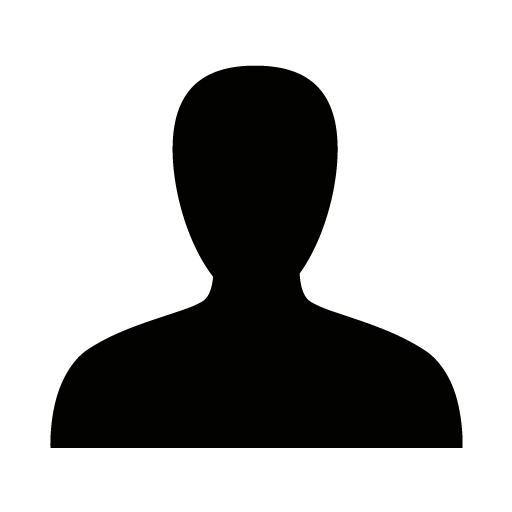
Nuclear Magnetic Resonance (NMR) plays a critical role in understanding structural and dynamical disorder, and ionic transport diffusion pathways in solids, complementing diffraction or impedance spectroscopy methods. Here, we present a recently published approach[1] combining experimental and computational Magic Angle Spinning (MAS) NMR aimed at elucidating the local configurational disorder and oxide ion diffusion mechanism in promising structural families of fast oxide ion conductors having the melilite,[1] [2] langasite[3] and perovskite[4] structures.
Layered tetrahedral network melilite possessing the La1.54Sr0.46Ga3O7.27 composition exhibits the flexibility required to accommodate interstitial oxide anions leading to excellent ionic transport properties at moderate temperatures[2]. 17O and 71Ga MAS NMR spectra display complex spectral line shapes that could be accurately predicted using a computational ensemble-based approach to model site disorder across multiple cationic and anionic sites, thereby enabling the assignment of bridging/non-bridging oxygens (Figure 1) and the identification of distinct gallium coordination environments. The 17O and 71Ga MAS NMR spectra of La1.54Sr0.46Ga3O7.27 display additional features that are not observed for the parent LaSrGa3O7 phase[submitted] and that can only be detected at the most powerful NMR magnet in the world operating at 35.2 T.[5] These features are attributed to interstitial oxide ions incorporated upon cation doping and stabilised by the formation of five-coordinate Ga centres conferring framework flexibility.[2]
17O high temperature (HT) MAS NMR experiments capture exchange within the bridging oxygens at 130 °C and reveal coalescence of all oxygen signals in La1.54Sr0.46Ga3O7.27 at approximately 300 °C (Figure 1), indicative of the participation of both interstitial and framework oxide ions in the transport process. These results, further supported by the coalescence of the 71Ga resonances in the 71Ga HT MAS NMR spectra of La1.54Sr0.46Ga3O7.27, unequivocally provide evidence for the conduction mechanism in this melilite phase.
Other related examples on langasite[3] and perovskite[4] highlight the potential of MAS NMR spectroscopy to enhance the understanding of ionic motion in solids.
Westminster-O1
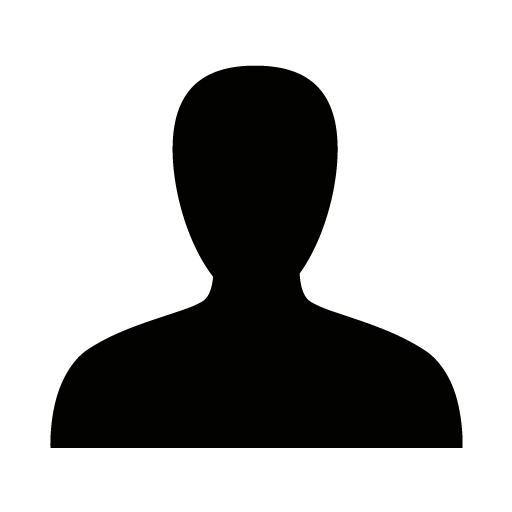
Solid state ionic (SSI) devices such as fuel cells, electrolyzers, batteries and sensors are becoming critically important in our effort to promote clean and renewable energy and decarbonize the future, due to their distinct advantages in efficiency and scalability over conventional conversion and storage technologies. Fast-ion conductors (FICs) are an indispensable component in SSI devices. The ionic conductivity of FICs plays a pivotal role in the performance of these devices. Known for their abundant, versatile defects and ion-transport-friendly environment, cubic perovskites have been enthusiastically explored in the past few decades as a promising class of FICs. In this presentation, we will first review recent advances in perovskite FICs. By combining theory and experiment, we will then discuss the interplay between defect-chemistry, ionic transport, and electrochemical stability using several cubic perovskite FICs, old and new, as illustrating examples. We will also highlight several useful experimental techniques to characterize ionic conductivity, transport number and electrochemical stability of FICs. We will finally provide our thoughts on potential applications of these perovskite FICs to generate new interest in developing innovative FICs and SSI devices.
Westminster-O2
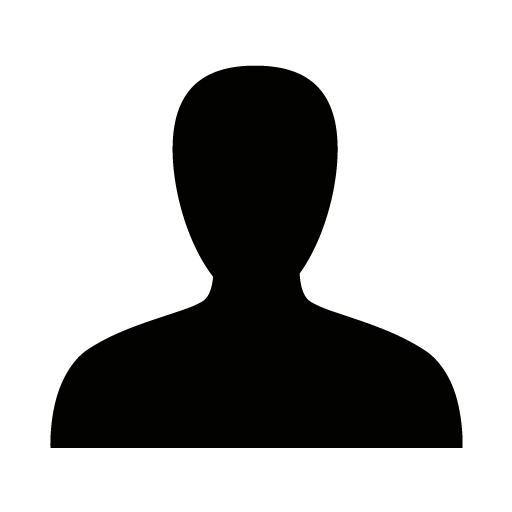
Solid oxide fuel cells (SOFC) have gained particular interest due to the demands for sustainable energy sources and reached a mature state of development. In contrast, there are still challenges in materials engineering for solid state electrolysis cells (SOEC). If an external electric current is driven through the cell in electrolysis mode, degradation effects due to electrochemical redox processes can occur, which may limit the lifetime. Electric current induced redox phenomena also gain importance for processing of ceramic materials using Field-Assisted Flash Sintering (FAST) and Spark Plasma Sintering (SPS). Intermediate reduction during the sintering process was recently reported. [1] Generally, when considering a contact between two mixed electronic and ionic conductors, electrochemical redox phenomena will occur, if the transference number of the electronic charge carriers is changing at the interface. This includes reduction phenomena as well as oxidative pore formation at electrode interfaces or in the interface between solid electrolytes and mixed conductors. [2, 3]
In this contribution we want to focus on the formation of blackened zirconia, known since the first reports by Casselton in 1968 and subject of various studies in recent decades. The cathodic electroreduction of yttria-stabilized zirconia (YSZ) will lead to a dendrite-shaped reaction front of a reduced phase moving to the anode side. In contrast to the solid electrolyte YSZ, reduced YSZ is a good electronic conductor, leading to an interface between reduced and unreduced phase with a large change of the electronic transference number. However, the composition and structure of the oxygen deficient phases is still not cleared in detail. In many studies it is reported that the mechanical properties of reduced single crystal or ceramic material degrades significantly (i.e. getting brittle).
Our new investigations on YSZ single crystals reveal strong strain fields leading to the formation of checkerboard-like structures on the surface above a highly oxygen deficient region below the surface. [4] Transmission electron microscopy (TEM), energy dispersive x-ray spectroscopy (EDX) and x-ray photoelectron spectroscopy (XPS) have been employed to understand its physical nature. The lattice constant of one cubic axes has shrunk significantly, Zr4+ is reduced to lower valences, resulting in an approximate stoichiometry of ZrO ∙ Zr2O. Moreover, there are many stacking faults in the reduced phase and misfit dislocations in the interface to the unreduced phase. The strong change of the molar volume may be the origin of the strain field resulting in the checkerboard-like surface structure and of the deterioration of the mechanical properties.
Westminster-O3
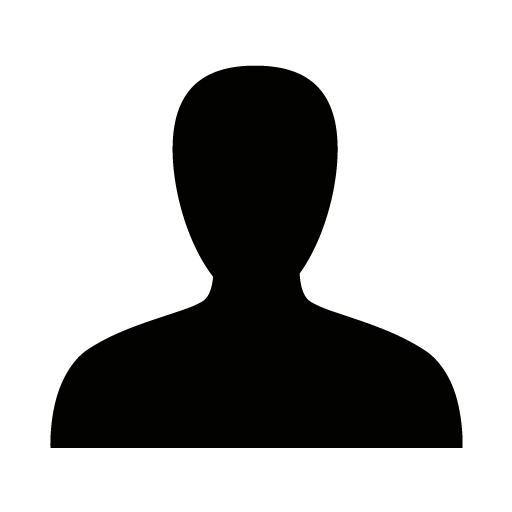
Improving the ionic conductivity of outstanding, composition-optimised crystalline electrolytes is a major challenge. Achieving increases of orders of magnitude requires, conceivably, highly non-linear effects. One known possibility is the use of high electric fields to increase the point-defect mobility. In this study, we investigate quantitatively a second possibility, that high electric fields can increase substantially point-defect concentrations. As a model system we take a pyrochlore oxide (La2Zr2O7) for its combination of structural vacancies and dominant anti-Frenkel disorder; and we perform molecular-dynamics simulations with many-body potentials as a function of temperature and applied electric field. Results within the linear regime yield the activation enthalpies and entropies of oxygen-vacancy and oxygen-interstitial migration, and from three independent methods, the enthalpy and entropy of anti-Frenkel disorder. Transport data for the non-linear regime are consistent with field-enhanced defect concentrations and defect mobilities. A route for separating the two effects is shown, and an analytical expression for quantitative prediction of the field-dependent anti-Frenkel equilibrium constant is derived. In summary, we demonstrate that the one stone of a non-linear driving force can be used to hit two birds of defect behaviour.
St.James-I1
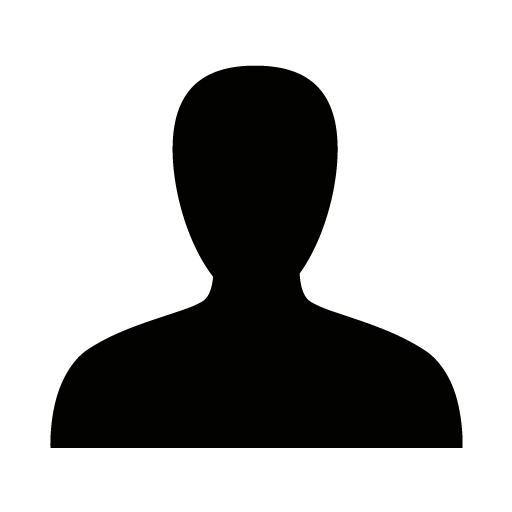
Protonic ceramic electrochemical cells (PCECs) offer significant promise as efficient and sustainable energy devices, capable of converting chemical fuels into electric power and vice versa, especially when operating at temperatures below 600°C. However, challenges arise due to the formation of secondary phases within perovskite electrolyte materials, stemming from harsh processing conditions involving high temperatures and prolonged annealing periods. These challenges contribute to a notable decline in the electrochemical performance of PCECs. Furthermore, PCECs encounter issues with poor catalytic activity at lower operating temperatures, resulting from sluggish kinetics at the air electrode. To address these obstacles, our research adopts an innovative approach that employs an ultra-fast sintering process, drastically reducing sintering times to just 5 min. Additionally, we introduce air electrodes based on newly doped BaCoO3-𝛿, strategically designed to enhance oxygen permeability and phase stability. These developments aim to improve the activity and durability of PCECs. In this presentation, I will discuss our recent progress in advancing highly efficient PCECs, highlighting our efforts to overcome these critical challenges.
St.James-O1
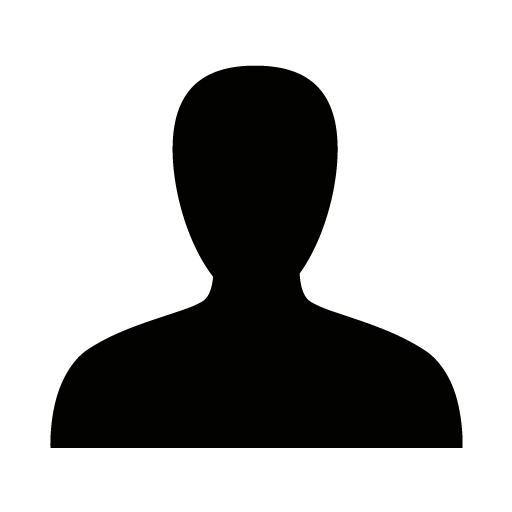
I study the positive electrodes (positrodes) of proton ceramic electrochemical cells - with particular focus on the water splitting reaction. Electrochemical impedance sectroscopy (EIS), Butler-Volmer theory, thermodynamics and DFT are some of my interests
Bulk protonic conduction may enhance the positrode performance for proton ceramic electrochemical cells by increasing the electrochemically active area beyond the triple-phase boundaries. Significant hydration and proton conduction is observed in positrode perovskites like lanthanum doped barium ferrite (BLF) where the B-site is acceptor doped with Ni and Zn [1], [2]. To further explore the properties as a positrode, electrochemical impedance spectroscopy (EIS) is performed on Ba0.95La0.05(Fe1‑x‑yNixZny)O3-δ (BLFNZ) deposited on proton conducting electrolyte Ba(Zr0.7Ce0.2Y0.1)O3-δ.
Various BLFNZ compositions are studied in a symmetrical cell with reference electrode. Key variables such as activation energies, pre-exponentials and predominant charge carriers are investigated by considering polarization resistance (Rp) as a function of pO2 and pH2O across a wide temperature range. Extracting individual area specific polarization resistances (Rp,i) at open circuit voltage reflects equilibrium conditions, while applying cathodic and anodic DC voltage to EIS sweeps delineate oxygen reduction- and oxygen ervolution reaction conditions, respectively. The voltage application results in a net current (I) through the cell and Rp,i is later converted into net overpotentials by integrating Rp,i over I.
This work combines EIS studies with thermogravimetric analysis (TGA) of Ba0.95La0.05(Fe0.7Ni0.2Zn0.1)O3-δ (BLFNZ10), amongst other BLFNZ compositions between 250-700 °C in varying pO2 and pH2O. The activation energy of 1.2 eV extracted at 350-450 °C in humid air (~3% H2O) is relatively high, but a small pre-exponential value (10‑7 Ωcm2) renders the Rp comparable to state-of-the-art positrode materials [3]. Assuming hydration dominates the mass change in humid air, TGA experiments reveal the proton concentration ranges from 1.8-0.03 mol% for 250-500 °C, i.e., sufficient for appreciable bulk protonic conduction. The relatively high activation energy may suggest a sluggish surface oxygen exchange reaction. Continued experiments include EIS with and without DC bias, and TGA experiments that may yield further insight to the reaction mechanisms of BLFNZ10. Thus, this study aims to establish relationships between hydration and the electrochemical properties of BLF based positrodes.
St.James-O2
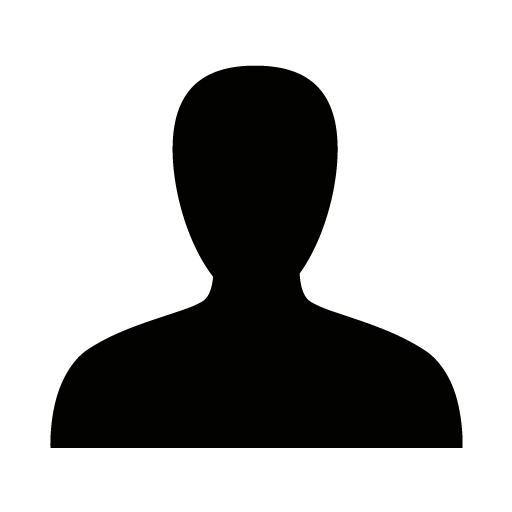
Proton ceramic electrochemical cells (PCECs) show promise for cost-efficient steam electrolysis, power generation, and membrane reactors. However, the air/steam electrode is a restricting factor in their performance, with the oxygen evolution and reduction reactions being rate limiting. A mechanistic understanding of the reaction steps at the electrodes is therefore required to further develop high-performance electrodes and cell architectures.
Pulsed Isotope Exchange (PIE) is a gas phase analysis method that allows for fast characterisation of the oxygen surface exchange rate of powder samples [1]. The surface exchange of oxygen is measured as a function of oxygen pressure and temperature, as well as the effect of water on the exchange rate. The reaction mechanisms and rate determining steps are investigated further by electrochemical impedance spectroscopy on model electrodes at temperatures from 500 °C down to 200°C in different pO2s and pH2Os.
Various compositions of Ba1-xGd0.8-yLa0.2+x+yCo2O6-δ, which are double perovskite type materials, have been shown to be efficient triple conducting air/steam electrodes for PCECs at intermediate temperatures [2, 3]. BaGd0.3La0.7Co2O6-δ shows an oxygen exchange limited by dissociative adsorption in all measured pO2s with activation energies ranging from 0.8 eV to 1.1 eV in dry atmospheres, which is comparable to other double perovskite cobaltites [4, 5]. While the presence of water does not change the rate limiting step, it lowers the exchange rate and increases the activation energy in high pO2 and increases the exchange rate and lowers the activation energy in low pO2s. Electrochemical impedance measurements show similar activation energies to PIE in the same atmospheres and temperature range.
St.James-O3
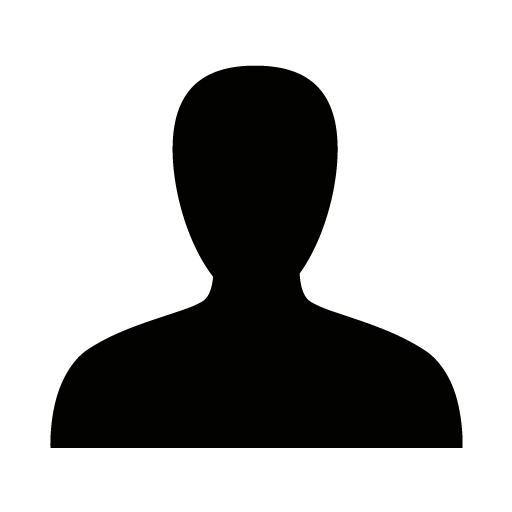
In the pursuit of advancing high energy density batteries, the incorporation of solid electrolytes as integral components in all-solid-state batteries (ASSBs) is a matter of significant interest. This solution may enable the next-generation batteries with better performances. In addition, it targets to replace the flammable liquid organic electrolyte used in current Li-ion technology in safe electric vehicles that complies with a net-zero world goals. [1]
Among different types of solid-state electrolytes, halide-based inorganic materials have gained the remarkable attention in the battery field. They are known to have high ionic conductivities, compatibility with high-voltage cathode materials and wide electrochemical stability windows. Herein, we focus on lithium indium chloride (Li3InCl6) that presents ionic conductivity values exceeding 1 mS/cm at room temperature. [2] The combination of inorganic electrolytes and polymers in hybrid solid electrolytes (HSEs) makes possible their processing in the current battery manufacturing lines. [3, 4]
Our study is based on Li3InCl6 electrolyte and different non-conducting polymers such as styrene-ethylene-butylene-styrene (SEBS) and polyisobutylene (PIB) in order to get flexible and self-standing thin membranes (< 30 μm) with a high inorganic content (58 vol. %). This work aims to understand the ionic conduction transport across the halide-based HSEs but also boost the design and fabrication of advanced materials suitable for Li-metal all-solid-state battery applications. For this, we study the effect of SEBS and PIB polymers on the Li-ion transport properties in the hybrid electrolyte. This research includes electrochemical impedance spectroscopy (EIS) the inorganic-polymer interface characterization, which reveals the significant impact of the polymer on the ionic conductivity properties. Moreover, we investigate dependence of the stack pressure on ionic conductivity and Li+-diffusion in the system by EIS and PFG NMR measurements.
Moore-I1
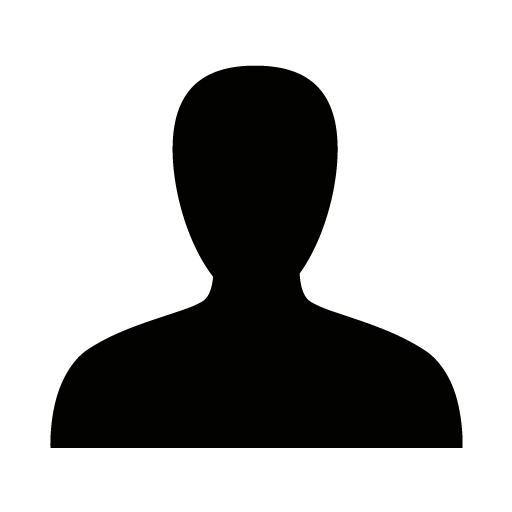
Raman spectroscopy is a non-destructive vibrational spectroscopy technique which offers valuable insights into the structural characteristics of materials by using scattered light to measure the vibrational energy modes of a sample. As the vibrational frequency of a specific mode is inversely proportional to the effective reduced mass, its wavenumber is sensitive to the mass of the atoms constituting the molecule or the crystal, and varies for different isotopes. It is thus possible to determine the concentration of a specific isotope in a material and to measure its spatial or temporal variations by carrying out in situ Raman measurements.
The Isotope Exchange Raman Spectroscopy (IERS) technique is based in the continuous measurement of a specific Raman mode of a given material (such as an oxide) while it is exposed to an isotope enriched atmosphere (e.g. 18O) within a temperature-controlled environment. It allows for the estimation of the isotopic concentration as a function of time (and/or position), facilitating the measurement of ion transport kinetics, including oxygen surface exchange and diffusion coefficients, in functional materials. This innovative approach was first developed for gadolinium-doped ceria (CGO), a well know ionic conducting oxide typically used as an electrolyte in solid oxide cells.[1] For thin films we demonstrated that employing various sample configurations allows for the in situ measurement of both in-plane and out-of-plane kinetic coefficients. In addition, the IERS technique has been further developed and expanded to the study of several mixed ionic electronic perovskites thin films, but also to bulk materials and composites. During my presentation, I will discuss the latest findings, as well as the advantages and limitations of the technique.
Moore-O1
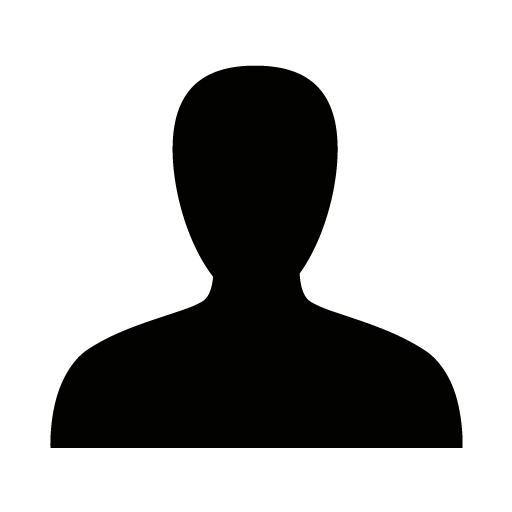
Solid oxide electrolysis and fuel cells (SOEC/SOFC) have drawn much attention due to their outstanding efficiency and superior fuel flexibility [1-3]. However, a significant challenge in scaling SOEC/SOFC technologies is the degradation of cells during operation. To address this, advanced operando characterization needs to be developed, which may offer insights into the mechanisms behind cell degradation.
Electrochemical impedance spectroscopy (EIS) emerges as a powerful technique for the nondestructive investigation of degradation in the solid oxide cell, or its individual components [4]. Integrating EIS with operando transmission electron microscopy (TEM) allow us to perform detailed structural and compositional analyses during EIS testing. In previous research, we successfully achieved analysis of pure gadolinia-doped ceria (CGO) material in relevant gas environments, at elevated temperatures, and under electrical polarization inside a TEM, and introduced the EIS-TEM methodology. This was achieved by combining an environmental TEM (ETEM) with a heating-biasing holder and a special sample preparation procedure [5, 6].
Based on previous works, this study employs the EIS-TEM methodology to investigate a model solid oxide cell composed of CGO electrodes and an yttria-stabilized zirconia (YSZ) electrolyte. The cell was fabricated using pulsed laser deposition (PLD) followed by focused ion beam scanning electron microscopy (FIB-SEM) processing. We determined the activation energies for YSZ ion transport and CGO surface reactions to be 0.9 eV and 0.5 eV, respectively, which are in close agreement with the literature. This study demonstrates the viability of conducting direct SOEC/SOFC cell tests within a TEM, including EIS analysis during operation, thereby providing profound insights into the reaction mechanisms of the cell.
Moore-O2
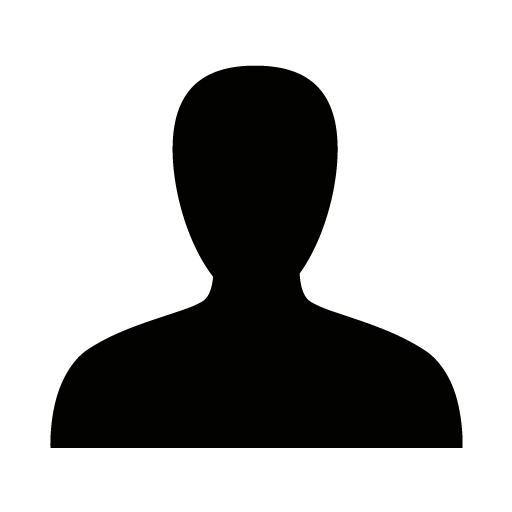
Finding the optimal mixed conducting materials for use in Solid Oxide Cells (SOCs) or catalytic applications requires detailed knowledge of the electrochemical charge transport properties and oxygen exchange kinetics. These properties are governed by the defect chemistry of the material and cation segregation phenomena, which both are very closely linked to the oxygen stoichiometry under various temperature, atmosphere and ageing conditions.
Here, we present two new, fast and precise cell types for coulometric titration and quasi in-situ XPS measurements to investigate the bulk defect chemistry and link it to the surface chemistry of the mixed conducting perovskite Sr1-xTi0.6Fe0.4O3-δ with x ranging from 0 to 0.07. For powder samples, we use a miniature titration cell with dedicated pumping and sensing electrodes. Thin film samples deposited by Pulsed Laser Deposition (PLD) on YSZ were used in sealed oxide ion battery [1] type cells, on which oxide ions are directly exchanged by pumping through the electrolyte. The big advantage of coulometry over thermogravimetry is the much broader pO2 range that is accessible electrochemically, compared to gas mixtures. By running multiple oxidation and reduction cycles within and beyond the thermodynamic stability limit, we can differentiate between reversible oxygen stoichiometry changes and irreversible metal exsolution reactions, with corresponding phase transitions. This enables us to investigate differences in oxygen stoichiometry, thermochemical stability and kinetics of metal exsolution between materials on a fundamental level. For the thin film samples, these coulometric techniques were combined with simultaneous Electrochemical Impedance Spectroscopy (EIS) and in-situ X-ray Spectroscopy (XPS), thus quantifying surface stoichiometry, and linking transition metal oxidation states on the surface with bulk oxygen vacancy concentration determined from coulometry.
In summary, we could show that coulometric techniques and in-situ XPS are valuable techniques to characterise the bulk defect chemistry and surface reducibility of various oxide materials.
Moore-O3
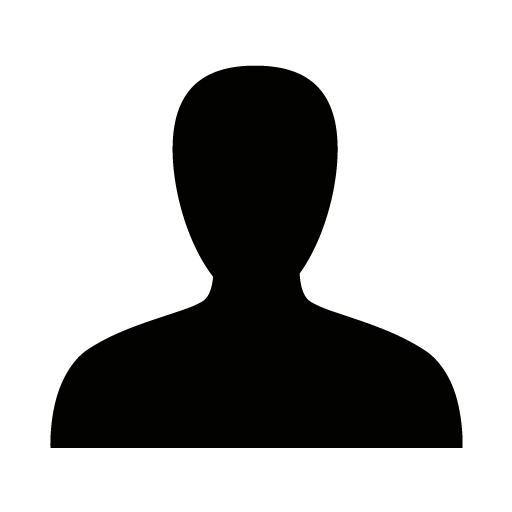
Solid oxide electrolysis cells (SOECs) represent a promising avenue for sustainable energy storage, conversion, and the synthesis of fuels and chemical components. While state-of-the-art nickel and yttria-stabilised zirconia (Ni|YSZ) cermet cathodes exhibit high efficiency for CO2 and H2O electrolysis, they are plagued by issues such as coking and morphological degradation. In contrast, ceria-based cathodes, such as nickel and gadolinium-doped ceria (Ni|GDC) cermets, and particularly single-phase GDC cathodes, emerge as compelling alternatives as the mixed conductivity of GDC under reducing conditions leads to a high electrochemical activity. A major benefit of especially single phase GDC cathodes is their high coking resistance during CO2 electrolysis. However, the notable drawback of ceria-based electrodes lies in their pronounced chemical expansion, causing mechanical stress, fractures, and potential cell failure. Furthermore, similar to Ni|YSZ, morphological transformations under intense electrochemical polarisation are anticipated for Ni|GDC due to solid-state (de)wetting phenomena, yet the intricate details of these transformations need further investigations.
In this study, our objectives are two-fold: (i) Investigate the (de)wetting behaviour of nickel on YSZ and GDC (ii) Unravel the intricacies of the chemical expansion observed in GDC under electrochemical polarisation.
For our first topic, dense Ni thin film electrodes are sputter deposited onto bare YSZ single crystals or YSZ substrates that have previously had a GDC film grown using pulsed laser deposition (PLD). Employing techniques such as electron microscopy, focused ion beam cutting, and electron backscatter diffraction, we examine the wetting behaviour under varying atmospheres and polarisations. The data obtained were matched with a computational phase field model and thus form the basis for understanding and predicting the morphological changes in SOEC cermet electrodes.
To fulfil our second goal, single phase, PLD grown GDC thin film electrodes are investigated by the means of in-situ X-ray diffraction (XRD) to study their chemical expansion behaviour under electrochemical polarisation. Electrochemical impedance spectroscopy is employed to simultaneously obtain the chemical capacitance of GDC electrodes, which provides us with the minority point defect concentration related to the expansion. Correlating in-situ XRD results with the material’s defect chemistry, establishes the groundwork for tailoring the electro-chemo-mechanics of SOEC cathodes, that will be done in forthcoming doping-variation experiments.
Abbey-I1
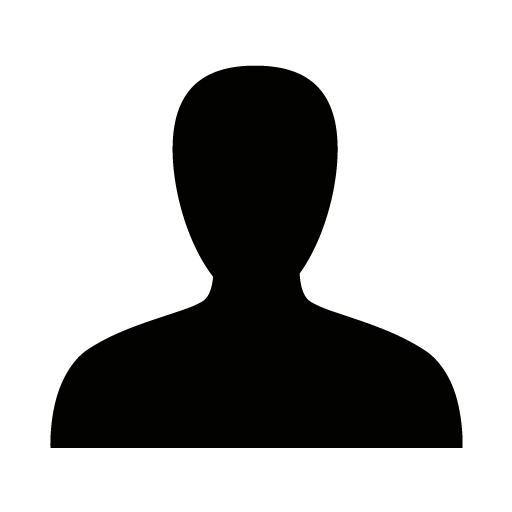
Improving battery safety is a top priority for consumers and battery manufactures. For that reason, a variety of approaches have been investigated to prevent thermal runaway, which is the uncontrollable accelerating heating of batteries that causes fires/explosions.
We have recently pioneered the use of ceramic materials, introduced inside the batteries, to achieve a fast and early shutdown of the electrochemical reactions triggering the thermal runaway [1]. The ceramic material was a La and Nb co-doped BaTiO3 whose resistivity drastically increased with temperature. As a result, when the battery heated under abuse conditions (overcharge and overheating testing), the ceramic material became insulating, thus effectively stopping the battery electrochemical reactions that release heat and induce further, escalating battery heating. Therefore, the introduction of this ceramic material can effectively complement other safety measures to produce batteries that remain safe even when they are operated outside their specifications.
Another approach to enhance battery safety is the implementation of solid electrolytes, which can be achieved with the help of a small amount of liquid electrolytes to facilitate materials’ contacts. Unfortunately, the interface between solid and liquid electrolytes associates a large resistance, which then compromises battery performance, but we have shown that the introduction of water in low concentrations as an additive effectively suppresses such interfacial resistance [2].
Abbey-O1
The demand for extended-range electric vehicles has created a renaissance of interest in replacing the common metal-ion with higher energy-density metal-anode batteries, i.e. lithium, sodium and zinc. However, metal cells suffer from capacity fading and potential safety issues due to uneven metal electrodeposition.
"Anode-free" (AF) batteries comprise a metal-ion cathode and a current collector, the cathode consisting of the metal source. The metal is then plated on the current collector during charging. These batteries present a significant advantage due to their higher energy density, superior safety, and ease of production. However, realising metal and AF batteries requires a better understanding of alkali and multivalent metal plating, short-circuiting mechanisms, and metal battery degradation.
A considerable performance gap between lithium symmetric cells and practical lithium batteries motivated us to explore the correlation between the shape of voltage traces and degradation. The coupling of operando nuclear magnetic resonance (NMR) and galvanostatic electrochemical impedance spectroscopy (GEIS) allowed us to observe metal batteries' electrochemical and chemical dynamics and degradation in real-time without affecting the electrochemical reactions in the cell. [1-2]
"Soft shorts" are small localised electrical connections between two electrodes that allow the co-existence of direct electron transfer and interfacial reaction. Although soft shorts were identified as a potential safety issue for lithium-ion batteries in the early nineties, their detection and prevention were not widely studied. Using coupled EIS and operando NMR, we showed that transient (i.e., soft) shorts form in realistic conditions for battery cycling.
The typical rectangular-shaped voltage trace, widely considered ideal, was proven, under the conditions studied here, to be a result of soft shorts. Recoverable soft-shorted cells were demonstrated during a symmetric cell polarisation experiment, defining a new type of critical current density: the current density at which the soft shorts are not reversible. We showed that soft shorts are predictive towards the formation of hard shorts, demonstrating the potential use of EIS as a relatively low-cost and non-destructive method for early detection of catastrophic shorts and battery failure while demonstrating the strength of operando NMR as a research tool for metal plating in metal batteries. [1-2]
While today's lithium-ion battery chemistries are ubiquitous, powering mobile devices and increasingly electric vehicles, they contain critical minerals such as cobalt and minerals with supply concerns, mainly if these batteries are used at the scale needed to meet the 2050 climate goals. To address this challenge, "beyond-lithium" technologies are investigated. These battery chemistries allow earth-abundant, safer, and low-cost energy storage.
Sodium metal anodes have been studied broadly, assuming sodium and lithium metal anodes respond similarly to cycling. We established that lithium and sodium short circuit formation mechanisms fundamentally differ and strongly depend on electrolyte composition and SEI stability.
Zinc metal anodes have gained increasing interest due to the sustainability of the aqueous electrolytes; however, a greater insight into their unique plating, hydrogen evolution, and corrosion mechanisms is critical.
We demonstrated how SEI composition heterogeneity determines lithium plating morphology. [3] These findings inspired us to explore scanning electrochemical microscopy (SECM) methods for electrochemical mapping of the SEI. In our SECM study of zinc plating, we compared the effects of various electrolyte compositions and plating conditions on SEI heterogeneity and zinc metal morphology.
This new understanding of the metal plating mechanism is crucial to developing the next generation of rechargeable batteries with high energy density, prolonged cycling life and improved sustainability. A fundamental understanding and reliable testing methods for soft short circuits are critical for commercialising metal (e.g., metal-air, metal-sulphur) and AF batteries.
Abbey-O2
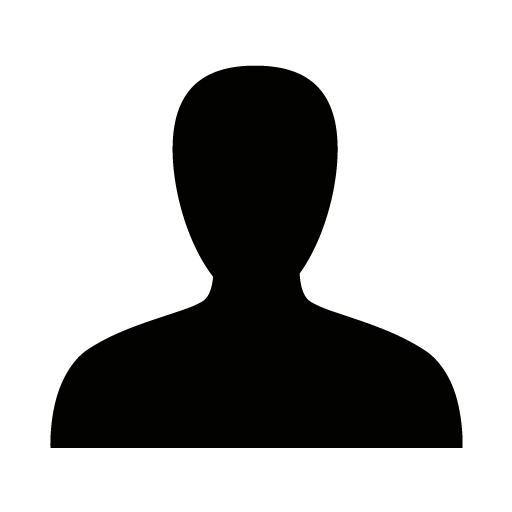
The use of inorganic ceramic solid electrolytes (SEs) to produce all-solid-state lithium batteries (ASSLBs) can overcome the safety issues related to conventional liquid electrolytes and enable Li batteries to operate at higher voltage. To make this happen, one of the most crucial requirements for the SE is to possess a large electrochemical stability window (ESW). Li7La3Zr2O12 (LLZO) solid electrolyte has attracted a lot of attention given its high ionic conductivity and because it was first reported with a very wide ESW (0–9 V vs. Li+/Li). It was not until recently that this value of the ESW was challenged, mainly proving that the oxidation of LLZO happened at a much earlier potential than 9 V. In the same line of thought, our work describes a methodology to accurately determine the ESW of doped M:LLZO (M = Al, Ta and Nb). The ESW of M:LLZO was found to be identical for all three dopants and much narrower than previously documented, on both reduction and oxidation sides. The reduction reaction was evidenced through ex situ 7Li nuclear magnetic resonance and operando powder X-ray diffraction analyses.
Abbey-O3
Dr. Jongwoo Lim currently serves as an Associate Professor of Chemistry at Seoul National University. His academic journey began at POSTECH, where he obtained his undergraduate degree in Chemistry in 2008. He later pursued his Ph.D. in Chemistry from the University of California, Berkeley, graduating in 2013 under the guidance of Professor Peidong Yang. Post-Ph.D., Dr. Lim engaged in postdoctoral research with Professor William Chueh at Stanford University, before joining the Department of Chemistry at Seoul National University in 2017 as an Assistant Professor.
Batteries undergo both active cycling and prolonged idle storage throughout their lifespan. While degradation mechanisms induced by cycling and their mitigation techniques have been deeply studied, the specific effects of storage without cycling remain largely underexplored. Notably, battery performance also sees a unique decline over time, contingent on the state-of-charge (SoC) when the batteries are at rest. Capacity decline during SoC70 storage primarily arises from electrode slippage and Li inventory loss in a full cell. This is accompanied by a minor structural breakdown of Ni-rich layered oxide cathodes. Conversely, SoC100 storage leads to a more pronounced structural impairment of Ni-rich cathodes and pronounced side reactions. Intriguingly, these severe side reactions curb the Li inventory loss, electrode slippage, and the reduction of full-cell capacity during SoC100 storage. In addition to standard degradation processes, such as Li/Ni cation mixing, the formation of surface reconstruction layers, and the emergence of exhausted phases, cathodes stored at SoC100 displayed an unexpected contraction of interlayer spacing during post-storage cycling, highlighting the atypical effects of storage. Based on the mechanisms of capacity reduction highlighted in this study, we propose strategies to counteract the aging caused by storage. This research offers valuable perspectives for refining battery production and management to enhance their calendar lifespan.
Gielgud-I1
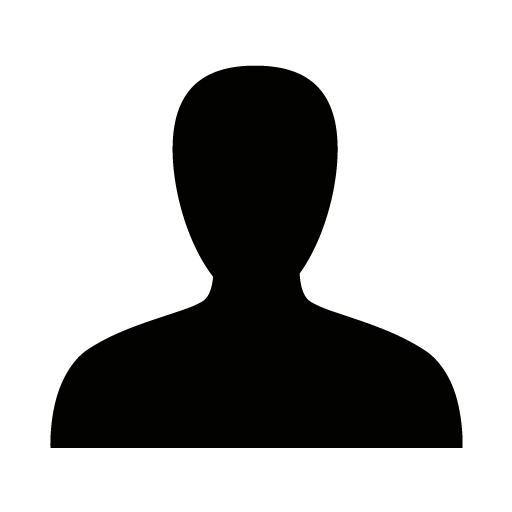
High temperature fuel cells (SOFC) are efficient and clean energy generation systems.
An important characteristic of these devices is that they can operate in reversible mode,
acting as electrolyzers (SOEC) for the generation of hydrogen. The use of surplus
electricity from renewable or continuous sources of electricity allows the production of
so-called green hydrogen (and oxygen), making them a key element in reducing the
carbon footprint of the global energy system. In this sense, these cells also allow,
through the coelectrolysis of CO2 and water, the production of other fuels widely used
industrially for the production, among others, of synthetic natural gas.
Achieving efficient and durable operation at low fabrication and operation costs is the
main challenge for the implementation of large scale high temperature electrolysis. We
explore here different strategies to reach this goal. On one hand, the optimization of air
electrodes is crucial in order to allow high density currents and we deal with the
addition of nanocatalysts on conventional LSM electrodes. On the other hand, we
explore the potential of the operation under pressurized conditions in order to increase
the efficiency through the decrease of the overvoltage contributions at cell level and
ease the integration on the whole system. Microtubular geometries overcome the poor
mechanical behaviour of flat membranes while facilitating easier sealing.
Key Words: Solid oxide fuel cells; Hydrogen production
Gielgud-O1
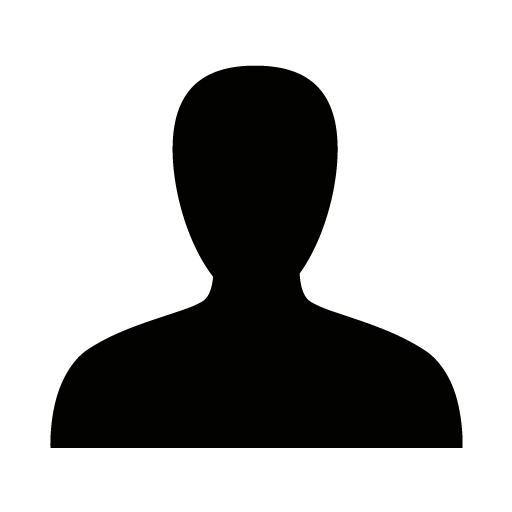
A solid oxide fuel cell (SOFC), an eco-friendly energy conversion device, has gained significant attention as a power generator due to its high fuel flexibility and efficiency and low emissions of pollutants. However, SOFC has inherently poor thermomechanical stability, ascribed to ceramic properties, such as high elastic modulus and low thermal conductivity. Therefore, it is prone to crack formation and failure of the cell in the thermal shock caused by rapid temperature changes, leading to slow start-up/shutdown and unstable operation under thermal cycling. To achieve exceptional thermomechanical strength, an innovative design of the solid oxide cell is indispensable, thereby overcoming the inherent vulnerability of ceramics to thermal shock.
This study examines the temperature and stress distribution in a solid oxide cell exposed to rapid heating conditions via computational simulation. Thermal stress analyses were employed to assess the thermomechanical robustness of anode-supported and electrolyte-supported SOFCs. Critical vulnerabilities within each SOFC configuration were identified, providing essential insights into their respective thermomechanical stabilities.
Gielgud-O2
Dr. Rinlee Butch Cervera is a full professor and laboratory head of the Energy Storage & Conversion Materials Research Laboratory at the Department of Mining, Metallurgical and Materials Engineering and the current program coordinator of the Energy Engineering Graduate Program, College of Engineering, University of the Philippines Diliman. His research specialization and expertise are on energy storage and conversion materials and devices such as fuel cells, hydrogen, and Li-based batteries, waste-to-energy research, and recycling or materials recovery of waste/spent Li-ion batteries. He received his Bachelor of Science, Master of Science, and Ph.D. degrees, all in materials engineering from UP Diliman, Universiti Sains Malaysia, and the University of Tokyo, Japan, respectively, and a postdoctoral research fellowship at the National Institute for Materials Science (NIMS) and the University of Tokyo, Japan. Dr. Cervera was a Balik-Scientist Awardee of the Department of Science and Technology (DOST) in 2012, UPD Balik-PhD awardee, an Outstanding Young Scientist of 2018 by the National Academy of Science & Technology (NAST), the 2018 Professional Degree Awardee for Materials Engineering by the UP Alumni Engineers, 2019 Outstanding Engineering Researcher and 2021 Outstanding Engineering Professor of the College of Engineering UP Diliman, an awardee of the David M. Consunji Award for Engineering Research by the Philippine Association for the Advancement of Science and Technology (PhilAAST) in 2022, a Young Affiliate Awardee of The World Academy of Sciences (TWAS), a UP Scientists 3, and 2023 100 Asian Scientists Honoree by ASM. Dr. Cervera is also the current Editor-in-Chief of the Philippine Engineering Journal (PEJ).
For the next generation energy conversion and storage systems, the development of fuel cells and electrolysis cells is an important endeavor to achieve a sustainable and clean alternative energy source in support of the global sustainable development goals. Thus, R&D activities in developing green and reliable energy storage and conversion technologies are at the heart of successful incorporation in the power mix and securing global future energy needs. One of the research efforts at our laboratory focuses on development of a reversible solid oxide electrochemical cells (SOEC), from materials considerations to device fabrication. In this presentation, new material electrode composite compositions and synthesis methods such as for LSM-ScYSZ, Ni-ScSZ, and highly conducting solid electrolyte material of Sc and Y co-doped ZrO2 have been investigated and its electrochemical performance in a solid oxide fuel and electrolysis cell applications will be reported. For Sc and Y co-doped ZrO2, the XRD, Raman and PES analyses revealed the complementary structural characteristics of the material while SEM/EDS results showed the morphological and compositional analyses. The Sc and Y co-doped ZrO2 solid electrolyte thin film also revealed a high total conductivity of about 2.8x10-1 at 700 oC. In addition, I-V curves revealed the reversible performance of an electrolyte-supported fabricated single cell (5x5cm2) in both the fuel cell and electrolysis cell modes.
Gielgud-O3
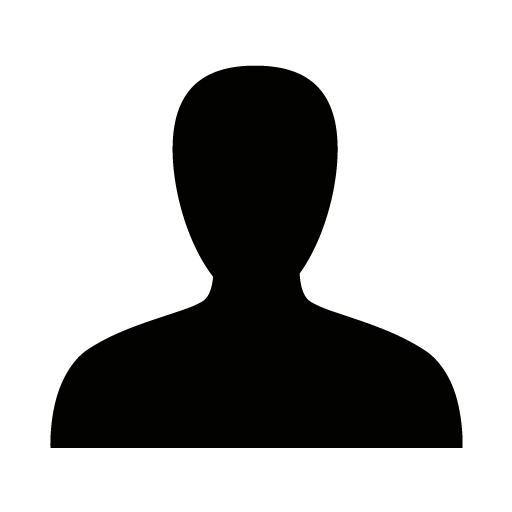
Solid oxide electrolysis technology is gaining attention for its potential in clean synthetic fuel production. However, electrode degradation remains a significant issue, particularly in the microstructure change of the Ni-YSZ fuel electrode. This study involved the fabrication of Ni-YSZ cross-sectional model electrodes, with the morphological changes of the fuel electrodes being quantitatively evaluated by laser microscopic observations under operating condition. To evaluate the microstructure evolution of Ni/YSZ fuel electrodes, comb-shaped symmetric Ni pattern electrodes were designed on the top layer of the YSZ thin film. When operated in fuel cell mode, one side of the Ni pattern electrode spreaded, resulting in an increase in triple phase boundary (TPB) length and a decrease in electrolyte width. On the other hand, when operated in electrolysis mode, the Ni pattern electrode slightly migrated away from the electrolyte. To prevent electrochemical oxidation, platinum electrode was used as a counter electrode. Then, quantitative analysis of the Ni microstructure change in electrolysis operation became possible.
Fleming-K1
In order to realize the promise of superior energy density, the solid electrolytes in solid-state batteries have to synergize fast ionic conductivity with a low contribution to the overall cell mass. While the dead mass of the “separator” solid electrolyte layer can be minimized by reducing its thickness to the extent limited by operational safety and processability, the catholyte within the inevitably thick composite cathodes will constitute 80-90% of the total electrolyte volume in high energy density cells. Thus, in the absence of fast-ion conducting high energy density cathode materials, low density catholytes are of key importance to maximize the energy density of all-solid-state batteries. Additional constraints for fast-ion conductors include electrochemical and electromechanical compatibility as well as interface wettability with the electrode materials. Soft, compressible electrolytes not only facilitate densification, they also increase both the effective interfacial contact area and prolong cycle life by retaining close interfacial contact with breathing electrodes.
Here we discuss two design strategies to realize such compressible low-density fast-ion conductors. The first strategy involves ultrathin ceramic-in-polymer Composite Solid Electrolytes (CSEs). We developed CSEs combining Li-doped polyacrylonitrile with various ceramic electrolytes (Li1.5Al0.5Ge1.5(PO4)3, LiTa2PO8 etc.) with and without plasticizers. Optimized composites reach Li+ conductivities of 0.1 – 1 mS·cm-1 at densities < 2 g cm-3 and retain wide electrochemical windows. Temperature-dependent analysis of Li+ paths shows that the ceramic filler induces a percolating network of fast ion-conductive interphases below the glass transition of the bulk polymer. Interfacial resistance is drastically reduced by spin coating and in situ UV-curing the precursor slurry on Li anode and composite cathode, respectively. Symmetric cells cycled with up to 2 mA·cm-2 over 500h retain a stable overpotential and no dendrite growth is observed. LFP/CSE/Li cells achieve a maximum specific discharge capacity of 148 mAh·g-1 and retain 89% of that after 200 cycles. Stable room temperature cycling is demonstrated for cells with a cathode mass loading of 6 mg·cm-2. Strong binding and three-dimensional architecture of the in situ-formed interfaces is crucial for the favourable cycling stability.[1]
An alternative all-ceramic design strategy is to exploit the correlation between fast ionic conductivity, density and glass forming ability in glass-ceramic oxides[2] and oxyhalides[3]. The higher number of monovalent light halides required to balance the charges of typically heavier high valent glass-former cations reduces the overall density and turns the material less brittle. Compared to close-packed halides, oxyhalides typically have lower coordination numbers, creating free volume for ionic motion. In view of their favorable oxidation stability yet limited reduction stability, oxyhalides are in particular suitable to function as catholytes. The recent report[3] of fast room temperature ionic conductivity exceeding 10 mS cm-3 in LiMOCl4 (M = Nb, Ta) exemplifies the strong potential of the so far hardly explored class of crystalline oxyhalides. Our redetermination of LiNbOCl4 (density 2.7 g cm-3) finds a simpler and more plausible, Li-disordered, tetragonal crystal structure with smaller unit cell than the originally reported orthorhombic phase. The revised structure model [4] is consistent with DFT simulations. Molecular dynamics simulations utilizing our bond-valence related embedded-atom method type forcefield softBV-EAM reveal the ion transport mechanism, the origin of the temperature dependent activation energy and its relation to the rotational mobility of (NbOCl4–)n polyanions therein. The simulations thus indicate that LiNbOCl4 is in a plastic-crystalline state at room temperature, which explains its favorably high compressibility and formability of LiNbOCl4.
Fleming-I1
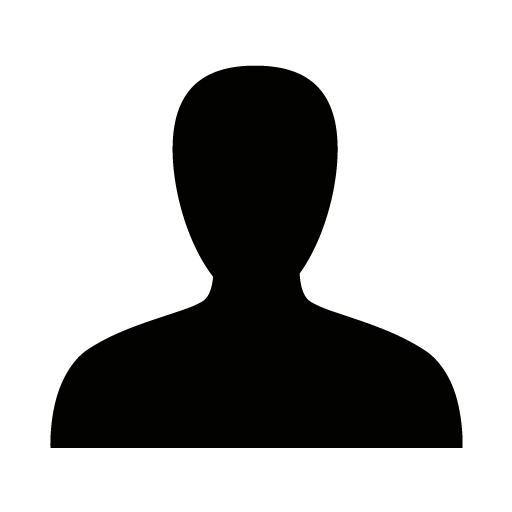
Li-La-Ti-O (LLTiO) is one of the promising electrolytes for all solid-state batteries, and a number of studies on LLTiO and related oxides have been reported in last decades. It has been known that LLTiO electrolyte has various ordered structures in different microscopic scales: LLTiO has an ordered crystal structure along the c-axis at the atomic scale, whilst there are microdomains composed of those ordered crystal structures. The ordering of microstructure significantly could affect the diffusion and mechanical properties of LLTiO. The present study based on ab-initio calculation and molecular dynamics elucidates the deformation behaviour and Li-ion diffusion mechanisms of LLTiO with various orderings of microstructure. In addition, the Li-ion diffusion behaviours under various conditions of mechanical stress are investigated. The microstructural analysis are performed and also the energy barrier for Li-migration is discussed, considering directional deformations and properties. The results reveal unique deformation and diffusion behaviors of LLTiO of ordered microstructures, which are attributed to different lattice deformations between La-poor and La-rich layers and over domain boundaries.
Fleming-O1
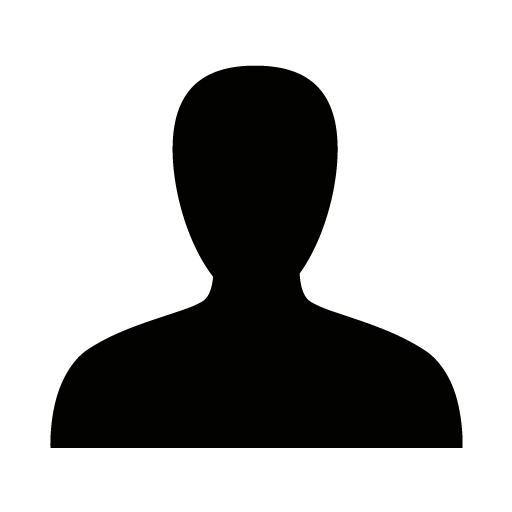
Recently, halide materials have emerged as potential inorganic electrolytes for all-solid-state lithium batteries (ASSLB) thanks to their moderate-high ionic conductivity at room temperature and their high-voltage wide electrochemical stability compared to other solid-state electrolytes such as polymers or sulfides, allowing halides to be electrochemically compatible with 4V cathode materials [1]. On another hand, their high ductility and the low-moderate temperatures needed for their synthesis make halides ideal candidates for the upscaling of material preparation, stacking and densification steps on cell manufacturing. Nevertheless, halides must overcome some challenges that might prevent their use such as the stability with the lithium (Li) metallic anode. In contact with Li, the halide undergoes a reduction reaction, leading to the formation of a mixed ionic-electronic SEI [2] until either the lithium electrode or the halide is consumed. As such, understanding the ionic transport and the Li/solid electrolyte interface dynamics is essential to optimize halide-based ASSLB.
In the present work, we report the advantages and the potential of Li3YCl4Br2 (LYCB) halide as solid-state electrolyte. First, LYCB presents a high ionic conductivity at room temperature, greater than 1 mS cm-1, which can be further improved after a densification step at moderate temperature. The cycling of full cells with a high-voltage NMC622 cathode, LYCB and metallic lithium anode leads to a discharge capacity up to 150 mAh g-1. Using computational methods, impedance spectroscopy measurements and physico-chemical characterizations, the dynamics at the Li/LYCB interface are evaluated. An increase of the interfacial resistance with time witnesses the formation of a resistive interface between the halide and the Li electrodes. With a good agreement, both simulations and experimentations demonstrate the reduction of LYCB by the Li. However, the tests performed suggest that the evolution of the Li/LYCB interface due to the reactivity between the two components leads to the formation of a SEI which is beneficial to the cycling performances with Li electrodes, enabling the stripping and plating of a symmetric cell during 1000 h applying a current density of 0.1 mA cm-2 with a capacity of 0.5 mAh cm-2. The cells show an overpotential as low as 40 mV, one order of magnitude lower compared to other halides in contact with Li electrodes [3,4]. Post-mortem analysis evidence the formation, during cycling, of a multi-layer interface, made of the resulting products issued from the reduction of LYCB, that might protect LYCB from further degradation by the Li electrodes. Overall, this study highlights LYCB as a promising candidate for use as electrolyte Li-metal solid-state batteries.
Fleming-O2
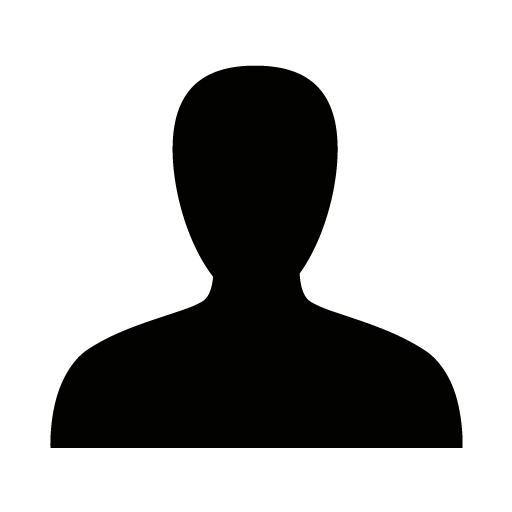
All solid-state batteries are a promising technology due to their employment of inorganic solid electrolytes (SEs), which are safer compared to their flammable, liquid counterparts. Recently, halide SEs have gained significant attention due to their relatively high ionic conductivity (> 1 mS/cm), wide electrochemical stability window, and compatibility with various electrode materials. However, prior to 2023, no known halide materials could reach the conductivities of the state-of-the-art sulfide SEs (> 10 mS/cm). This can be attributed to the close-packed anion arrangement of existing halide conductors, which confines lithium ions to narrow and high-energy conduction pathways. Recently, a new Li oxyhalide superionic conductor LiMOCl4 (M=Nb/Ta) was reported with extremely high ionic conductivities greater than 10 mS/cm [1]. Unlike previously found halide conductors, LiMOCl4 has a non-close-packed anion framework that provides a large conduction pathway. We present a first-principles investigation of the phase stability, electrochemical stability, and Li-ion conductivity in the LiMXCl4 (M = Ta, Nb, Sb, Ti, Zr, Hf, Sn, and X = O, F, Cl) family of superionic conductors. We identify a mixed-anion framework as key to stabilizing the oxyhalide structure and demonstrate the impact of aliovalent anion and cation substitutions on material properties. Long ab-initio molecular dynamics simulations spanning 10’s of ns confirm room temperature superionic conductivity in all systems. Probabilistic analysis of the rotational dynamics at the picosecond timescale contributes direct, quantitative evidence correlating the high Li-ion conductivity in oxyhalide systems with the soft tilting motion of MO2Cl4 octahedral groups present in the material. Our findings supply the theoretical underpinnings for the effectiveness of LiMXCl4 family of superionic conductors as catholytes compatible with 4-V-class layered cathodes and provide essential design criteria for constructing new Li halide superionic conductors with conductivity greater than 10 mS/cm.
Fleming-O3
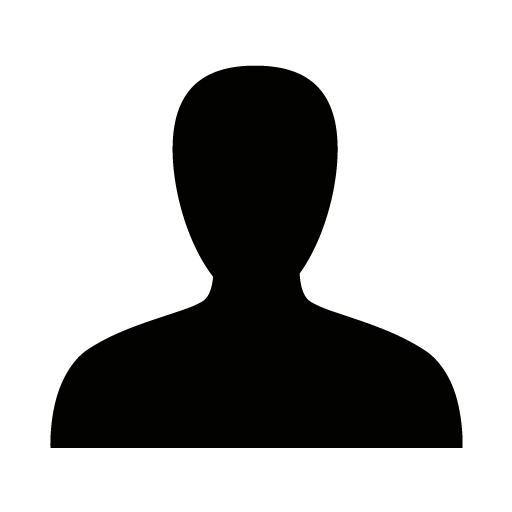
Among the different solid electrolytes, lithium halides show promising ionic conductivity and cathode compatibility. The complex composition-properties relationships makes rational design very challenging. In the first part of this work use the ionic potential, the ratio of charge number and ion radius, can effectively capture the key interactions within halide materials, making it possible to guide the design of especially complex halide compositions. This is demonstrated by the preparation of a family of complex layered halides that combine an enhanced conductivity with a favorable isometric morphology. In the second part we evaluate the the role of the Cl vs Br anion in the Li3YBrxCl6-x halide and investigate the trade-off between ionic conductivity and electrochemical stability. One of the main limitations of this material, and in general halide solid electrolytes, is the electrochemical instability at low working potentials, limiting its application. In this context we study the electrochemical and structural transformation and their (ir)reversibility, providing more insights into the stability limits of this material.
Westminster-K1
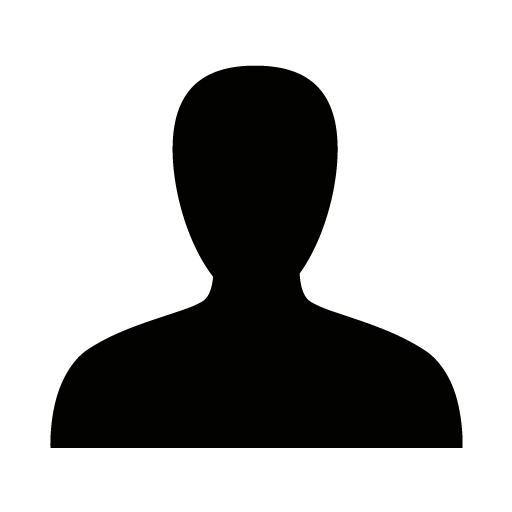
Advanced techniques for structural and microstructural characterization of materials are powerful tools to understand their fundamental properties. In the chase for higher resolution to understand the atomic origins of functional properties of oxides, scanning transmission electron microscopy (STEM) provides substantial advantages. However, in the cases under study, basic crystallography prior to the use of these advanced techniques is mandatory to completely solve the crystal structure of the materials. The electron diffraction (ED) patterns reflect the unit cell symmetry. The relations between the reciprocal and real space, by interpretation of the ED patterns in combination with the corresponding TEM images, permit to conclude the basic crystallographic parameters. The combination of high angle annular dark field STEM (HAADF-STEM) images, also called Z-contrast images, and the electron energy loss spectroscopy (EELS) mapping from single atomic columns selected from the image, allows the locations of the cations (the heaviest atoms) in the crystal structure highlighting compositional modulations, while images taken in annular bright field mode (ABF-STEM) reveal the positions of the lighter atoms of the materials, the oxygen atoms in the case of oxides.
The crystal structure of a variety of (A/A’)nBnO3n−δ oxides with electrocatalytic properties for oxygen reduction reaction (ORR) has been solved by ED, HRTEM, STEM (HAADF and ABF modes) and EELS mapping [1], [2], [3], [4], [5], [6]. Strong relations between the ordering of the A cations, the coordination polyhedra around the B atoms and the location of the anion vacancies, with the properties and experimental behaviour of these materials are established. The nature of the B atoms highly affects the oxygen content and location of anion vacancies within the crystal structure, which deeply impact on the electrochemical properties of the materials.
Westminster-I1
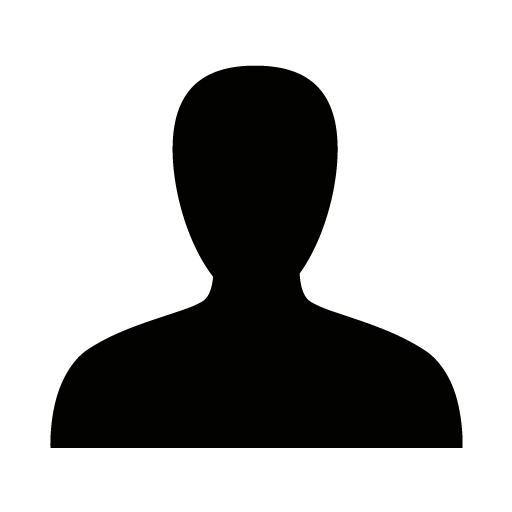
Complex oxides are renowned for their diverse functionalities that are essential to the advancement of electronics, energy, and information technologies. These functionalities include for example, ferroelectricity, piezoelectricity, and pyroelectricity. The extraordinary physical properties of these materials originate from the complex interactions among lattice, orbital, charge, and spin dynamics. In this talk, I will present our most recent research, focusing on how we can manipulate the properties of oxide heterostructures by intentionally altering their symmetry. A significant part of my presentation will be dedicated to exploring the advancements in freestanding oxide membranes. These have emerged as a groundbreaking platform for experimentalists to design materials with novel properties. Mainly made up of transition metal oxides, these membranes are produced as ultrathin, quasi-2D layers and can be reassembled into multilayered structures, allowing for precision in controlling the twist angles between layers. I will also introduce a pioneering method for creating high-quality complex heterostructures integrated with Si, using SrTiO3 membranes as a universal platform. This aspect of our study paves a new way for embedding electronic devices with multifunctional physical properties into silicon-based technology, promising significant advancements in the field.
Westminster-O1
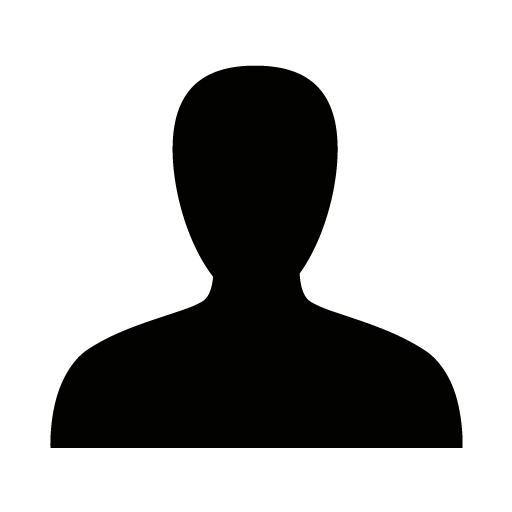
Electrochemical control has emerged over the last decade as a powerful approach for developing reprogrammable microelectronic devices. Such oxide based devices are characterized by remarkably large changes in chemical composition at low energetic cost. Both from a fundamental science and applications-oriented perspective, the ability to control the functional properties of metal oxides dynamically through their chemical composition opens a broad perspective in developing new classes of microelectronic devices. While such concepts have been demonstrated, important questions remain about how to optimize their device performance best. Information regarding ionic transport within the oxides remains elusive given the challenge of isolating it from the dominant electronic conduction. Moreover, many current device concepts not only rely on the transport of ions within a single oxide layer but across interfaces with additional layers as well. Adequate methods for studying the ionic properties in these ultra-thin films and isolating their transport kinetics are lacking. Their development will be vital for mastering overall device performance, such as speed, retention, and predictability.
To address these challenges, we have been investigating a model system capable of reversible ion transport and exchange under an applied field between two adjacent thin film solid oxide layers. Our investigations have focused on studying a PrxCe1-xO2/La2-xCexCuO4 bilayer stack that utilizes model materials for which the defect chemical and transport properties have previously been carefully characterized, that offer high ionic mobilities and can accommodate large levels of non-stoichiometry [1,2]. Building on our previous observations that we could reversibly modulate the resistance of the stack by over an order of magnitude through the transfer of ionic species, we discuss how our conductivity results can be interpreted via defect chemical models and show how such transfer can be tracked in situ inside a TEM by monitoring local valence and lattice dimension changes. Furthermore, we demonstrate that we can isolate the ionic transport kinetics, both within and across the solid oxide interfaces, by a combination of DC bias impedance measurement and dynamic current-voltage studies. This allows us, for example, to systematically study how the ionic mobility varies in PrxCe1-xO2 as a function of stoichiometry, as controlled by the degree of ionic titration. The findings in this work can be expected to aid in developing material selection and design criteria for similar bilayer systems and be used to achieve faster resistance switching speeds, larger resistance switching ranges, and longer device retentions.
[1] Tuller, H. L., Bishop, S. R., Chen, D., Kuru, Y., Kim, J.J. & Stefanik, T. S. Praseodymium Doped Ceria: Model Mixed Ionic Electronic Conductor with Coupled Electrical, Optical, Mechanical and Chemical Properties. Solid State Ion 225, 194–197 (2012).
[2] Kim, C. S. & Tuller, H. L. Fine-Tuning of Oxygen Vacancy and Interstitial Concentrations in La1.85Ce0.15CuO4+Δ by Electrical Bias. Solid State Ion 320, 233–238 (2018).
Westminster-O2
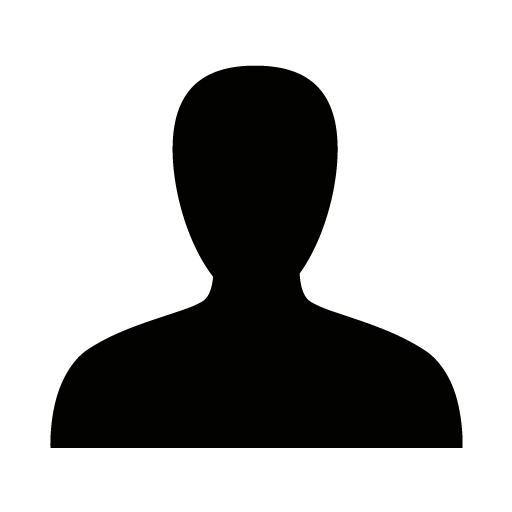
Mixed ionic and electronic conductors (MIECs) are key components for a vast number of electrochemical devices, e.g. as intercalation electrodes, sensors or permeation membranes. The functionality of the components rely on the ability of the material either to store or to transport the neutral species, i.e. the electrons as well as the ions. However, most materials with a high ionic conductivity exhibits a poor electronic one and vice versa. A common approach of dealing with this challenge is the preparation of artificial mixed conductors by mechanical mixing of a good electronic conductor with an ion conductor to prepare transport pathways for both electrons and ions. Consequently, these artificial MIECs are characterized by a high number of interfaces, which may significantly affect charge storage and charge transport in the composites. A detailed understanding of the impact of the nanostructure and, in particular, electron-conducting/ion-conductor interface on charge transport and charge storage properties of the composites is essential for the design and the improvement of electrochemical devices with improved functionality. However, the number of systematic studies to address this question is still scarce. Motivated by recent results regarding the mixed-conducting properties of mesoporous CeO2/YSZ nanocomposites [1], we present the preparation and characterization of multilayer heterostructures formed by ceria (CeO2) and yttria-stabilized zirconia (YSZ). The multilayers were prepared by pulsed laser deposition to achieve thin film structures with well-defined interfaces. To identify the impact of the interfaces on charge transport, samples with constant thickness but varying number of interfaces were deposited. Structural characterization was performed using Raman microscopy, X-Ray diffraction analysis, scanning electron microscopy and time-of-flight secondary ion mass spectrometry. The electrochemical properties were characterized using electrochemical impedance spectroscopy at different temperatures and under varying atmospheric conditions.
Westminster-O3
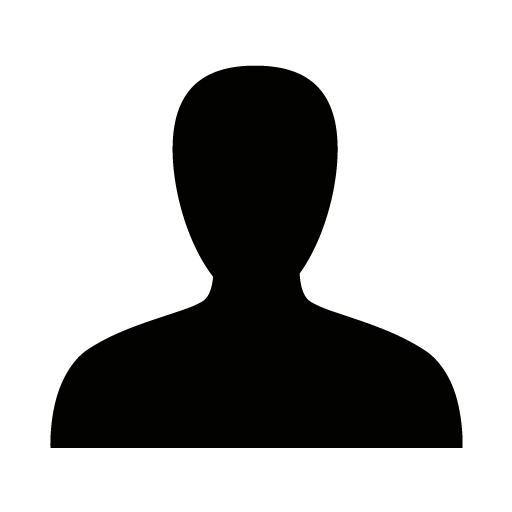
In the wake of global efforts to meet climate change goals, solid-state ionic conductors are receiving increasing interest as promising materials for electrochemical applications. One crucial feature of solid-state ionic conductors is high ionic conductivity. Enhancing ionic conductivity would allow a lower operative temperature while guaranteeing higher currents and lower overpotentials, making the material a good candidate for superior fuel cells or battery applications. Ionic conductivity follows an Arrhenius-type law, suggesting an increase in conductivity with decreasing activation energy. However, decreasing activation energy is also typically correlated with orders of magnitude decrease in the pre-exponential factor, which is known as compensation law [1]. Previous designs of ion conductors have struggled to overcome such compensation law due to a lack of fundamental understanding and descriptors (tunable design variables). Therefore, a deeper understanding of the processes governing ionic conductivity and fundamental descriptors to overcome compensation law could enable superior designs of solid-state ionic conductors.
In this presentation, we discuss designs of solid-state ion conductors with low activation energies and high pre-exponential factors. Using density functional theory simulations on perovskite oxygen ion conductors, we highlight that the entropy of migration regulates changes in the pre-exponential factor across many orders of magnitude. We uncover that such entropy of migration is the result of changes in the vibrational structure of the local environment of oxygen atoms occurring from equilibrium to the transition state during ion hops. Based on this understanding, we highlight strategies and descriptors to enable high pre-exponential factors by enabling the softening of the local environment of mobile ions during migration. On the other hand, we recently showed that the local electronic structure of lattice point defects regulates the migration barrier, which can be decreased by increasing the charge screening capability of the local host lattice [2]. Therefore, independently tuning the vibrational structure of the local environment of mobile ions and the electronic structure of lattice point defects can open a new design space to overcome compensation law. This framework is tested on perovskites and other crystal structures and mobile species. Our new findings and proposed descriptors hold great potential for accelerating the discovery of superior solid-state ion conductors leveraging emerging characterization techniques or growing approaches of computation-aided designs.
St.James-K1
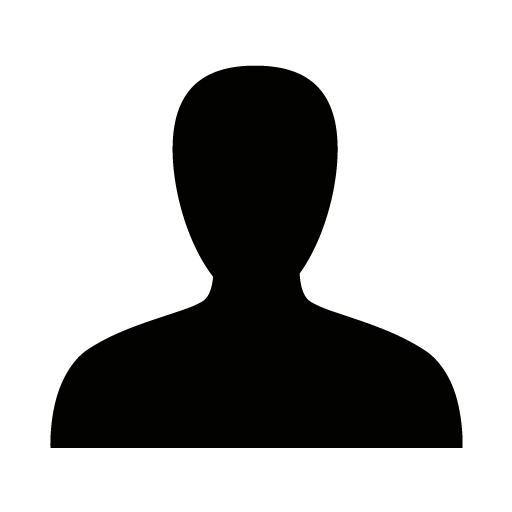
Despite their relatively simple stoichiometries, compounds in the barium–group(V) oxide series Ba4M2O9 (M = V, Nb, Ta) adopt three distinct structure types depending on composition and synthetic conditions: a completely unique and complex γ phase; [1,2] a uniquely distorted 6H-perovskite; [3] and a partially disordered α phase related to Sr4Ru2O9-type. [4] They all hydrate to very high degrees of up to 1/3 (γ) or 1/2 (6H and α) H2O per formula unit and show appreciable mixed oxide/proton conductivity. Intriguingly, none of these structure types contain oxide vacancies that would explain their hydration and conductivity in terms of a conventional hydroxylation mechanism, nor layers for intercalation. We have used diffraction, electron microscopy, physical property measurements, NMR, inelastic neutron scattering and DFT calculations to show how water is incorporated into the crystal lattices in ways that depend upon and guide their temperature-dependent structural distortions. The potential significance of these results extends beyond their ionic conductivity to the Earth sciences, because even at ppm levels, the pressure- and temperature-dependencies of lattice hydration processes mean that they affect the total amount of water available on Earth and the rheological properties of the lithosphere (plate tectonics, earthquakes etc.).
St.James-I1
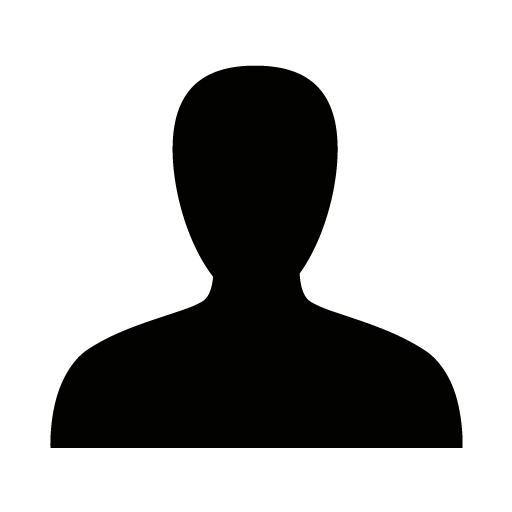
Ionic conducting materials are the most important parts in electrochemical devices such as batteries, fuel cells and electrolysers. Different types of ionic conducting materials, which conduct O2-, H+ or OH- ions, have been widely used in solid oxide fuel cells (SOFCs), solid oxide electrolytic cells (SOECs), proton exchange membrane fuel cells (PEMFCs) and electrolysers (PEM electrolysers), anion exchange membrane fuel cells (AEMFCs) and electrolysers (AEM electrolysers). The typical materials are solid oxides such as doped ZrO2 or CeO2 with fluorite structures or doped BaCeO3 (BCO)/BaZrO3 (BZO)/LaGaO3 (LGO) with perovskite structures1-10. The representative high temperature proton conductors based on doped perovskite oxides BCO/BZO were discovered in 1980 and 1990s respectively2, 6, while the high temperature O2- ion conductor based on doped LGO was discovered in 19943, 11. Those materials have been applied in SOFCs and SOECs. The typical operating temperature is above 550 °C while the durability of the solid oxide cells (SOCs) are not ideal due to the high operating temperature. When temperature drops, the O2- or H+ ionic conductivity in oxides is not high enough to provide high power density for the SOCs. Therefore it is desired to develop low temperature O2-, H+ or OH- ionic conducting materials.
Polymer membranes such as acidic proton-conducting Nafion membrane have been used in PEMFCs and PEM electrolysers but the acidic membrane are very expensive. Expensive noble electrode materials such as Pt, IrO2 have to be used in PEMFCs or PEM electrolyers leading to high cost, which is the key barrier for large scale applications. Also European proposes to ban all fluorine-based membrane such as Nafion in 2026-27. It is desired to find a replacement membrane for Nafion. The alkaline OH- ionic conducting membranes based quaternary ammonium groups have been investigated for over a decade but their stability is not ideal, also reacting with CO2 in air leading to reduced OH- ionic conductivity. It is urgent to discover new stable OH- ionic conducting materials for AEMFCs and AEM electrolysers.
Fortunately, in 2020, Tao's group at University of Warwick found that some ceramic materials exhibit high mixed OH-/H+ ionic conduction in water, with ionic conductivity exceeds 0.01 S cm-1 and ion transfer number above 98% at a temperature below 100 °C. Under certain conditions, the ionic conductivity exceeds 0.1 S cm-1. Some of ceramic OH- and H+ ionic conductors are very stable, ideal electrolyte materials for low temperature electrochemical devices such as fuel cells, electrolysers and batteries.
For the first time, ceramic low temperature OH- and H+ ionic conducting materials have been discovered. The application in direct ammonia fuel cells has been demonstrated as well.
St.James-O1
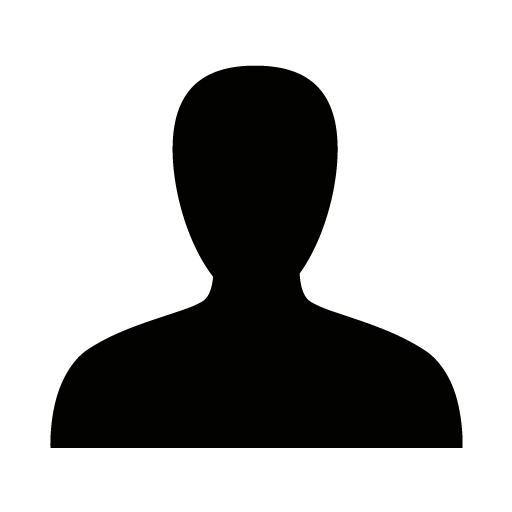
Mixed anion oxides, such as oxyhydrides and oxyhalides, showing exotic transport properties, are of interest in the research field of solid state ionics[1,2]. The anion-doping effects have also attracted much attention for perovskite-type proton conductors[3,4]. Meanwhile, unlike conventional cation-doping techniques, it is challenging for anion doping to precisely control and quantify the doping concentration, which is essential for interpreting their defect equilibrium. In this study, anion-doped Ba-Zr-based proton conductors were prepared by a topochemical reaction at high temperatures, and their defect equilibrium was clarified based on exact anion content analyzed by solid-state NMR spectroscopy and DFT calculations. The impacts of anion doping on the transport properties were also investigated.
Sc-doped, Co-doped, and (Sc, Co)-co-doped BaZrO3 were prepared as a single-phase host oxide by a solid-state reaction. BaF2, an F source, was mixed with the barium zirconate powders to have a molar ratio of 1:1. The topochemical reaction was carried out at 1200 °C for 10 h. The excess BaF2 that is unreacted can be removed by hot water after the reaction. 19F MAS NMR was conducted to identify the occupation site and amount of F ions introduced into the perovskite-type phase combined with shielding coefficients derived from DFT calculations.
19F MAS NMR spectra of undoped, Sc-, Co- and (Sc, Co)-doped BaZrO3 were taken after the topochemical reaction at 1200 °C. In addition to a BaF2 peak (-14.7 ppm), a new peak was observed at around -90 ppm for Sc-containing BaZrO3. The peak intensity increases with increasing Sc content. The DFT-calculated chemical shift of 2-coordinated F (Zr-F-Zr) in BaZrO3 was also found to be around -90 ppm, depending on local structures. Meanwhile, undoped BaZrO3 and Co-doped BaZrO3 show no extra peak other than BaF2. This NMR analysis implies that F can fully occupy O-sites of BaZrO3 to compensate for the effective charge of Sc, i.e., [ScZr’] = [Fo•], if enough F source is available at high temperatures. The F content in 5, 10, and 20%Sc-doped BaZrO3 was determined as 4.9, 10.2, and 17.1 mol%, respectively. Even though proton concentration in the F-doped samples was reduced due to the presence of Fo•, its impact on proton and hole conductivities strongly depended on the F content and the presence of Co. In addition to these F-doped samples, the defect equilibrium in hydride-ion(H-)-doped Ba-Zr-based oxides prepared by the topochemical reaction will also be discussed.
St.James-O2
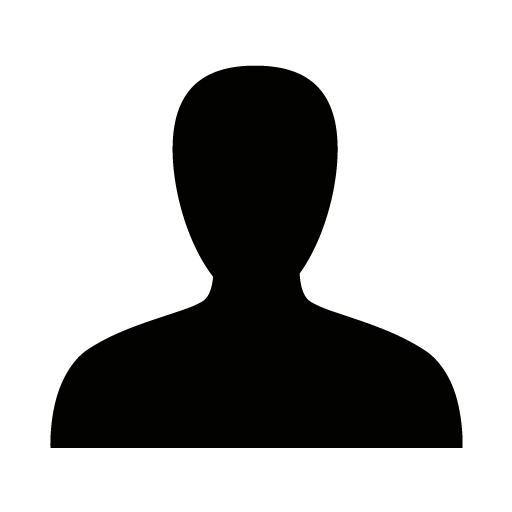
Structural features of materials, such as lattice distortions, water uptake, and oxide vacancies, are key factors that define protonic conduction. It is also worth noting that fast oxygen diffusion is advantageous for hydration kinetics since proton incorporation requires the migration of oxygen vacancies.
Here, a combination of 18O tracer isotopic exchange and Secondary Ion Mass Spectrometry (SIMS) measurements was applied to obtain oxygen surface exchange and diffusion coefficients of BaCe0.8Y0.1Yb0.1O3-δ (BCYYb), BaSn0.8Y0.1Yb0.1O3-δ (BSYYb), and BaZr0.8Y0.1Yb0.1O3-δ (BZYYb). The isotopic exchange was conducted in dry (18O2) and wet (H218O) atmospheres at various temperatures. Lower oxygen diffusion coefficients were observed for the wet exchange, indicating that the humidified and dry atmospheres affect oxygen transport differently. In some cases, variations in the surface exchange and diffusion coefficients were observed at the phase transition temperature, suggesting a different rate-limiting step in the oxygen exchange and diffusion processes. Oxygen diffusivity was correlated to the conductivity measured by impedance spectroscopy (EIS), and the equilibrium constants associated with water incorporation were derived from thermogravimetric analysis (TGA) in the temperature range 100-900 °C under a water partial pressure of 0.023 atm. The constants, calculated under the assumption of a negligible hole concentration, were linear in the Arrhenius plot. In contrast, high-temperature X-ray diffraction (XRD) revealed complex structural transitions. The structural changes linked to the water uptake were confirmed by Raman spectroscopy carried out at variable temperatures under dry and wet atmospheres. Results were in good agreement with data reported by Grimaud et al [1]. for BaCe0.9Y0.1O3-d, although the detection of OH bands using Raman spectroscopy remains an open discussion.
Moore-K1
Ifan is a Professor in Electrochemistry at the Department of Materials at Imperial College: he leads the Interfacial Electrochemistry Group there and is also Atoms to Devices Research Area Lead at the Henry Royce Institute.
Ifan joined Imperial College in July 2017. Prior to Imperial, he was at the Department of Physics at the Technical University of Denmark (DTU); he was first employed as a postdoctoral researcher, then as assistant professor and finally as associate professor and leader of the Electrocatalysis Group there. In 2015, Massachusetts Institute of Technology (MIT) appointed Ifan as the Peabody Visiting Associate Professor. He taught and conducted research at the Department of Mechanical Engineering at MIT for a whole semester.
Ifan’s research aims to enable the large-scale electrochemical conversion of renewable energy to fuels and valuable chemicals and vice versa. Such processes will be critical in order to allow the increased uptake of renewable energy. His focus is on the catalyst at the electrode, i.e. the electrocatalyst. It turns out that the electrocatalyst material defines the efficiency of several important electrochemical processes, including:(i) electrolysis for the storage of renewable electricity — which is inherently intermittent — in the form of fuels, such as hydrogen or alcohols.(ii) fuel cells as a potentially zero emission source of power for automotive vehicles. (iii) the green synthesis of valuable chemicals, such as ammonia and H2O2. (iv) batteries, which tend to degrade by gas evolution at the electrode-electrolyte interface. Hence the reactions that need to be accelerated in electrolysers and fuel cells — such as CO2, CO, O2 and H2 evolution — are precisely those that need to be inhibited in batteries.
Ifan has discovered or co-discovered several new catalysts for the oxygen reduction reaction, which exhibited significant improvements in performance over the prior state-of-the-art. In particular, his research on hydrogen peroxide production led to the establishment of the spinout company, HP Now.
Ifan is the recipient of RSC's Geoffrey Barker Medal (2024), the RSC's John Jeyes Award (2021). He also currently holds an European Resarch Council Consolidator Grant (2021-2025). Since 2022, he has been a Clarivate Highly Cited Researcher.
Interfacial reactions at the solid electrolyte interphase control the performance of batteries and electrochemical N2 and CO2 reduction. In batteries, degradation is typically accompanied by parasitic gas evolution reaction. In this contribution I will explore the commonalities between battery science and electrocatalysis and demonstrate the insight to be gained by studying different electrochemical reactions in parallel.
We recently demonstrated that on chip electrochemistry mass spectrometry - previously developed for aqueous electrocatalysis1 - can detect and quantify gases such as O2, CO2, C2H4 and H2 with ultra high sensitivity and time resolution during lithium ion battery operation.2 It allows us to probe degradation processes, which were previously unobservable. Augmented by 18O2 isotopic labelling, we reveal that lattice oxygen, not singlet oxygen is the more probable reactive species during the degradation of lithium nickel manganese cobalt oxide electrodes. We also show that CO2 reduction to ethylene takes place at graphite anodes.
Electrochemical nitrogen reduction to ammonia is a potentially sustainable and scalable alternative to the Haber Bosch process. Thus far, amongst solid electrodes, only lithium and calcium based electrodes in organic electrolytes can unequivocally reduce nitrogen to ammonia.3-5 Even so, at present, the lithium based system is far too inefficient and unstable for many practical uses. We hypothesise that the reactivity of lithium and calcium is due to (a) their ability to bind and dissociate N2 and (b) their ability to form solid electrolyte interphases that can moderate access to Li+ and H+ but facilitate access to N2.6 Herein, I will peruse upon these hypotheses, using a combination of electrochemical experiments, cryo-microscopy, infrared spectroscopy, electrochemistry mass spectrometry, time-of-flight secondary ion mass spectrometry, X-ray photoelectron spectroscopy and density functional theory On the basis of our insight, we propose new avenues towards going beyond lithium and calclium in electrochemical nitrogen fixation.
Moore-I1
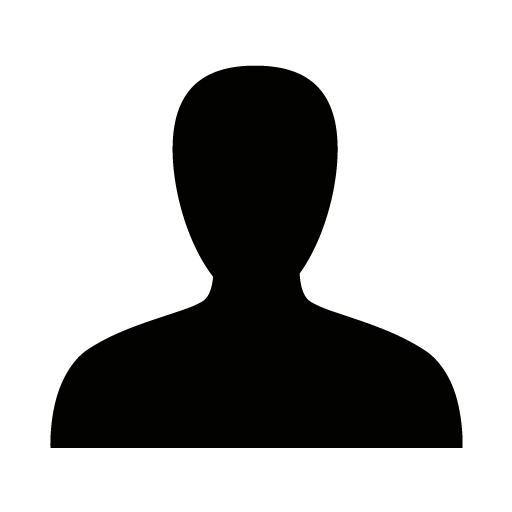
In Solid Oxide Fuel Cells, oxygen electrode polarization related to electrochemical reactions at the gas/solid interface is often the dominant flux limiting mechanism. The most common materials used as oxygen electrodes are metal oxides with mixed ionic and electronic conductivity. Their performance as an oxygen electrode is a function of the kinetics of exchange between lattice oxygen defects and molecular oxygen in the gas phase. As such, there has been tremendous efforts to characterize the rate of this oxygen exchange on mixed conducting oxides, with a wide variety of techniques.
One technique uses electrical conductivity as tool to track the stoichiometry changes within the oxide. With stepwise changes of oxygen pressure in the atmosphere, the stoichiometry goes through a transient state and stabilize to a value that equilibrate the oxygen chemical potential with that of the gas phase. This technique, called electrical conductivity relaxation, is usually performed on highly dense ceramics, and carries information of oxygen surface exchange kinetics as well as bulk ionic diffusion of oxygen defects. The latter can often be predominant in the total conductivity transient, which make the determination of surface exchange kinetics rates less accurate.
In a recent work1, we have introduced a variation of the electrical conductivity relaxation technique, which uses porous ceramics to improve the sensitivity to surface exchange processes. In this presentation, we will present this technique in-depth, and discuss the relevant parameters to consider for accurate measurements of surface exchange coefficients. The interpretation of relaxation profiles measured on porous ceramics requires an accurate description of the microstructure. The procedure proposed in this work features a simple determination of microstructural parameters from 2D images recorded by scanning electron microscopy. Then, those parameters are directly accounted for in the fitting procedure of the relaxation profiles with an adapted analytical model. The accuracy of the procedure is demonstrated on a wide range of mixed conducting oxides and microstructures.
Moore-O1
Juan Carlos Gonzalez-Rosillo obtained holds a M.Sc. in Materials Science and Nanotechnology and a PhD in Materials Science from the University Autonomous of Barcelona. He performed his MSc and PhD research (2011-2017) at the Materials Science Institute of Barcelona (ICMAB-CSIC), where he studied the relation of the resistive switching properties of metallic perovskite oxides with their intrinsic metal-insulator transitions for memristive devices and novel computation paradigms. He also was a visiting researcher at the University of Geneva (CH) and Forschungszentrum Jülich (DE). Then he joined the Massachusetts Institute of Technology (USA) for a postdoctoral position (2017-2020) working on the memristive properties of lithium-based oxides for neuromorphic computing and processing of next-generation solid-state electrolyte thin films for All-Solid-State Batteries and Microbatteries. Juan Carlos has been awarded with a Tecniospring postdoctoral fellowship to join IREC and to develop thin film microbattery architectures to power micro- and nanodevices for the Internet of Things revolution
Achieving facile, non-destructive chemical analysis techniques that can provide microscopic insights into the phase evolution of materials is essential for creating high-performance devices for energy harvesting and storage, among other applications. However, many of the most powerful techniques, such as isotopic ion exchange methods, in situ TEM, and synchrotron radiation-based techniques, are complex and limit easy access to crucial data.
Raman spectroscopy is a fast, non-destructive optical technique that provides quantitative chemical and structural information about the material under investigation. However, conventional Raman microscopy is diffraction-limited and unable to provide spatial resolution below ~ 1 µm. To overcome this limitation, we employ Tip-Enhanced Raman Spectroscopy (TERS), which combines the chemical sensitivity of Raman spectroscopy with high spatial resolution of scanning probe microscopy (SPM) and enables chemical imaging of surfaces at the nanometer length scale.
In this talk, we will discuss the fundamentals of TERS and its recent advancements in the implementation of the technique for electroceramic materials. We will showcase our latest results on Li-ion battery research using TERS, both ex-situ and operando configurations. The technique's spatial resolution capabilities will be highlighted, showing, for instance, the phase evolution at grain boundaries in battery cathodes with a resolution < 20 nm when cycled in an aqueous electrolyte.[1] We will also show our recent advancements in implementing operando TERS in battery cathodes with outstanding spatial resolution supported by experimental modeling emphasizing again the differences between grain and grain boundaries. Our efforts aim to demonstrate the versatility of TERS as a new approach for nanometric chemical insights of electroceramics in the energy field.
Moore-O2
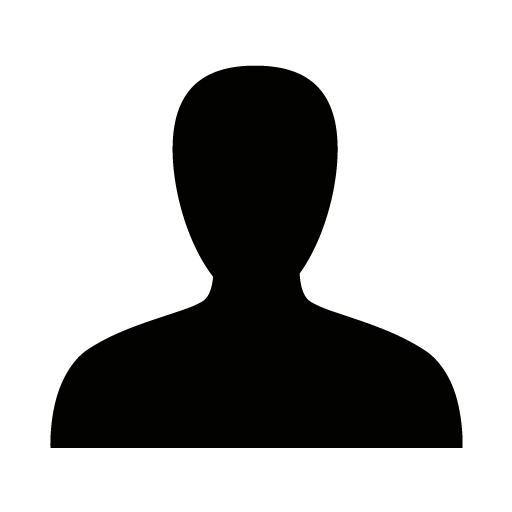
Since 2014, we have suggested a superior description of the impedance of the polycrystalline solid electrolytes [1-4] compared to the brick-layer model heavily stained by constant-phase-elements (CPEs) in most applications. Time- and painstaking parameter determination from the individual fitting analysis hindered further progress. The recent dissemination of Python-based data science tools and AI assistance like ChatGPT allowed the layman to code the algorithms that fit up to hundreds of spectra or thousands of impedance data labeled with temperature values. We applied the techniques to various Li7La3Zr2O12 (LLZ) samples. In essence, the AC response of the polycrystalline electrolytes can be described by the additive electric polarization relaxations, which can be represented by Havriliak-Negami (HN) capacitance functions or generalized Debye models, originating from the mobile charge carriers by the current constriction effects at the grain boundaries and also in bulk. The capacitance magnitude is inversely proportional to the temperature, and the time constants are activated similarly to the ionic conductivity. All the data are thus collapsed to the single trace of the complex capacitance. The overall sample resistance of the grain and grain boundaries is connected in parallel. Temperature-independent geometric capacitance with nearly constant loss (NCL) can be identified only at cryostatic temperature. Low-frequency blocking effects by the inert metal electrodes can be best described by the transmission line model (TLM) with the longitudinal resistance and the Warburg-interfacial impedance parameter activated by higher activation energy alike than the bulk one. While the interfacial and transverse shunt capacitance is inversely proportional to the temperature, as for the polarizations in the sample, the presence of the temperature-independent double-layer capacitance is also noted, which can be put at the other terminal of the TLM for the electrode response. The exponents of the Havriliak-Negami capacitance functions, similar to the power exponents of CPEs, can be and need to be fixed, unlike the conventional CPE modeling, the physics of which is related to the universal dielectric relaxation phenomena or Kohlraush-Williams-Watt stretched exponential functions. It has been found the low-frequency and high-frequency limiting exponents of one or half can be widely applied [5], which corresponds to the ideal relaxation and the diffusion-related relaxations, respectively. Havriliak-Negami capacitance functions (DE31) and the transmission line models with the generalized Havariliak-Negami capacitance elements (DX26) have been implemented in ZView® for the convenient preliminary analysis of the individual spectra. The models are directly indicated in the raw data presented in AC conductivity Arrhenius plots and capacitance and admittance Bode plots, and hence, are physics-based. The models can be successfully applied to the extremely nontrivial individual impedance of the composite solid electrolytes with the percolating conducting components for the battery electrode applications. Surface or near-surface conduction often leads to flattened activation at lower temperatures. The short-circuit paths as parallel resistance hardly affect the capacitance relaxations in the present modeling, so the original AC response and the surface conduction can be determined and separated.
Moore-O3
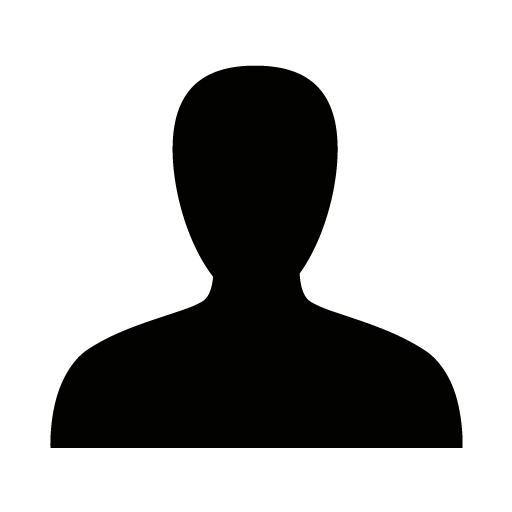
Ordered mesoporous oxides are characterized by a regular pore structure surrounded by single interconnected nanocrystallites. This unique architecture makes this class of material of high interest for a variety of electrochemical applications, e.g., for gas sensing, energy storage or catalysis. The open pore-solid framework provides good accessibility of the internal surfaces to the surrounding medium, as gases or liquids can completely penetrate into the mesopores, resulting in superior device performance compared to nanoparticles or disordered mesoporous counterparts. In addition, the electrochemical properties of ordered mesoporous oxides can be tailored, at least to some extent, by adjusting the pore size or by surface functionalization. However, a detailed knowledge about the impact of the mesoporous architecture on the electrical properties is necessary for further device optimization making a reliable electrochemical characterization of the materials indispensable. Electrochemical impedance spectroscopy is the method of choice to determine the electrical properties. However, the interconnected pore network hinders a simple estimation of the material-specific conductivity from the measured impedance.
Here, we use a 3D impedance network [1] to elucidate the impact of porosity on the impedance response of mesoporous thin films. The results demonstrate that the regular pore structure gives rise to a geometric current constriction effect, which dominates the impedance spectra in the whole frequency range. As a consequence, the effective conductivity values estimated from the total resistance and the electrode geometry underestimate the material-specific conductivity of the material by more than one order of magnitude. However, by a detailed analysis of computed impedance spectra for varying pore size, we were able to derive an empirical expression, which allows the estimation of the material-specific conductivity from the measured impedance with an error of less than 8% [2].
Abbey-K1
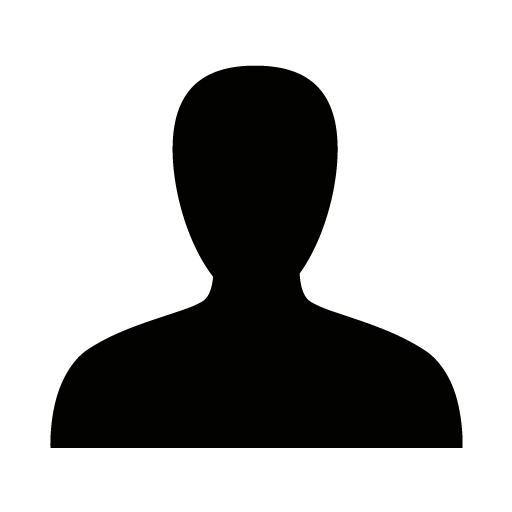
Rate capability is a limiting factor in solid oxide fuel cells (SOFCs), solid oxide electrolysis cells (SOECs), and oxide-based solid-state lithium (SSLiBs) and sodium (SSNaBs) batteries due to high area specific resistance (ASR). Lower ASR SOFC, SOEC, SSLiB, and SSNaB structures would enable higher power density and lower cost, dramatically improving market adoption of these energy conversion and storage technologies. In this presentation we will explain the roles of solid ion-conductor composition, structure, and interfaces in reducing ASR to achieve extremely high current densities. Results will be presented for GDC based SOFCs/SOECs, Li-garnet based SSLiBs, and NASICON based SSNaBs, and similarities between these dissimilar materials and electrochemical devices elucidated. Moreover, we will demonstrate that SOFC and SOEC current densities over 5 A/cm2 can be achieved at 650°C, and SSNaB and SSLiB current densities of 30 mA/cm2and 100 mA/cm2, respectively, can be achieved at room temperature, based on these similarities.
Abbey-I1
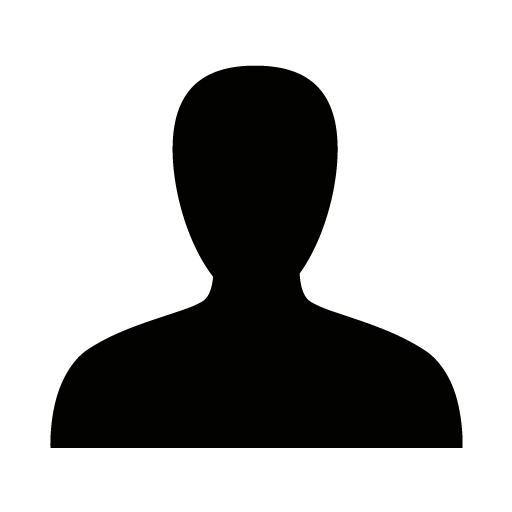
Some active materials used in lithium-ion battery (LIB) electrodes undergo phase separation into Li-rich and Li-poor phases upon lithium intercalation. Typical examples include LiFePO4 (LFP) at the cathode and graphite at the anode. While phase separation enables useful features, such as a constant equilibrium potential as a function of state-of-charge, such behaviour poses challenges during material characterisation and for high-rate operation.
This study provides a perspective of phase separation in LIB active materials by combining non-equilibrium thermodynamics principles [1] with in-operando techniques [2]. The results show that classical techniques used to estimate solid-state diffusion coefficients, such as the galvanostatic intermittent titration technique (GITT) [3], must be revisited for this class of materials. In fact, although the rapid equilibration of the interface between Li-rich and Li-poor phases within a particle leads to a quick voltage relaxation, the solid-state diffusivity can be significantly lower than what this fast dynamics may suggest. This has significant implications on the distribution of lithium within secondary particles because, upon fast lithiation, the Li-rich phase grows at the particle surface and prevents further lithiation. This is especially critical for graphite anodes, since the Li-rich phase (also known as stage I) at the particle surface is the primary cause for the plating of metallic lithium outside the particle, as quantified in in-operando experiments [2]. Nevertheless, experiments also show that Li plating is not irreversible and part of the plated lithium can be stripped and back-intercalated in graphite when the current is interrupted. This discovery opens opportunities for alternative fast-charging protocols as long as the particle size distribution is well controlled to prevent excessive plating on small particles. On the other hand, experimental characterisation and simulation of a disordered carbon, which does not undergo phase separation, reveal an effectively faster solid-state diffusion and a more significant resistance to lithium plating even at high C-rate [4], thus enabling for a comprehensive comparison between solid-solution and phase-separating active materials when it comes to characterisation and fast-charging capabilities.
Abbey-O1
Solid-state batteries using lithium metal anode has a huge potential as post lithium-ion batteries due to high energy density, safety, and fast charging capability. Lithium Lanthanum Zirconate oxide (Li7La3Zr2O12, LLZO) electrolyte is a promising candidate as it demonstrates an excellent compatibility with Li metal anode and notable performance in terms of their critical current density and cycling stability. Despite the promise, establishing a full “solid" cell architecture still faces challenges, mainly in achieving robust contact and low impedance for composite cathodes due to the formation of secondary phases. Thus, integrating with a choice of cathode material into practical devices has lagged, leading to the dearth of lab-scale prototype cell data. Here we report the electrical resistance of composite cathode, LLZO-LiCoO2, is reduced 3-4 orders of magnitude by manipulating sintering atmosphere. Raman mapping reveals the second phase of Li0.5La2Co0.5O4 are avoided in the composite cathode sintered in Li-rich atmosphere as compared to ambient condition. Practically thin electrolyte is fabricated either by co-sintering with the cathode composite or by pulsed laser deposition on the cathode substrate. Discussion is aimed toward further opportunities for all-ceramic cathode-supported cell development to meet important target parameters including interfacial resistance, cathode active loading, specific capacity, and cycling stability.
Abbey-O2
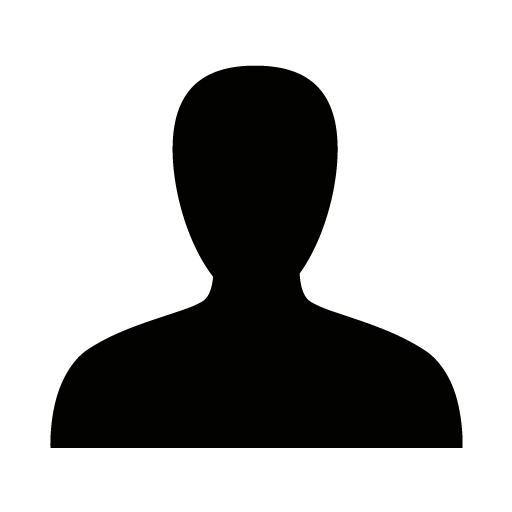
One of the most widely used electroanalytical methods for understanding the kinetics of composite battery electrodes is GITT – the Galvanostatic Intermittent Titration Technique [1,2]. At the same time, it is common for battery researchers to question the reliability of GITT measurements. The level of skepticism associated with GITT varies; some question its quantitative accuracy (but not much on otherwise trends), whereas others raise more serious questions on its relevancy for kinetics. The root causes for the unreliability of GITT are also perceived quite differently among researchers. Some believe that the unreliability is a result of poor implementation of the technique, implying that careful measurements could produce reliable results. Others believe that there are more fundamental limitations, dismissing any kinetic information obtained from GITT measurements. In this talk, we will isolate the implementation issues and focus on answering the question: "if the measurement protocol is optimized, can we extract relevant kinetic parameters from GITT measurements on composite battery electrodes?" We will take lessons from recent microscopic kinetics studies [3-7] and understand their implications from the perspective of using electroanalytical techniques such as GITT.
Abbey-O3
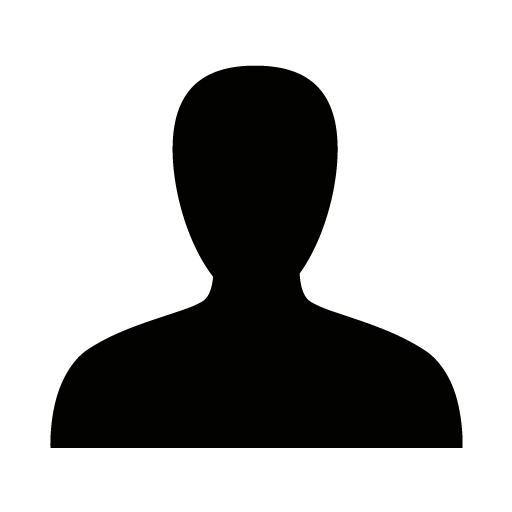
Inhomogeneous metal plating in batteries not only results in irreversible capacity losses, but also poses a serious safety concern for their operation. Dendritic growth of plated metal can cause internal short circuits and catastrophic failure of the cell [1]. Understanding the transport conditions that lead to heterogeneous metal deposition is essential for the development of a safer lithium-ion battery. Scanning Electrochemical Microscopy (SECM) is a scanning probe technique which is increasingly being used in battery research to characterise the heterogeneity of the solid electrolyte interphase (SEI), the transport properties of which directly affect the morphology of the plated metal [2]. Here we present a study linking the heterogeneity of an SEI to metal electroplating and battery performance using zinc as a model compound. Zinc is an ideal candidate for this study, as it has been shown to form an SEI and can be used in an aqueous electrolyte [3]. We present a comprehensive study demonstrating how different additives to the electrolyte affect the heterogeneity of the SEI and therefore impact metal electroplating within the cell. This research aims to provide a methodology to predict and prevent where inhomogeneous metal plating will occur and aid a strategy for mitigating dendrite induced failures.
Gielgud-K1
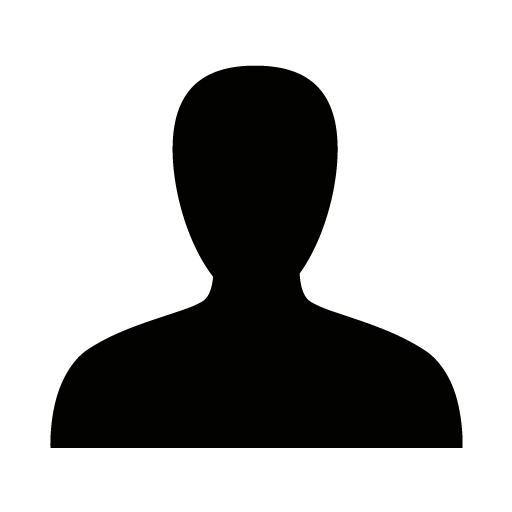
Solid Oxide Electrolysis Cells (SOEC) are solid-state devices that play a pivotal role in the efficient conversion of renewable electrical power into fuels, making them a key technology for the green transition. The SOEC technology allows for the production of green hydrogen through steam electrolysis, as well as the operation of SOEC in co-electrolysis mode (i.e., electrolysis of H2O+CO2) to generate synthesis gas (CO+H2). This gas can then be processed further into a variety of carbon-containing fuels.
To achieve high electrochemical performance and mechanical robustness of SOEC, it is essential to manufacture these ceramic solid-state devices to obtain a mechanically strong support layer, thin and gas tight oxide ion conduction electrolyte and porous electrodes having structures optimized to ensure the highest possible density of active sites for the electrochemical reactions e.g. large percolating triple-phase-boundary density in cermet-based electrodes like the often applied nickel/yttria-stabilized-zirconia based fuel electrode.
Advanced and complementary characterization techniques are needed for the further development and improvements of SOEC towards marked entry to ensure a high performing, reliable and long-term durable SOEC technology for production of green fuels. The characterization techniques include combinations of electrochemical characterization (e.g. impedance spectroscopy), element mapping (e.g. via EDS, Raman spectroscopy) and micro- and nano-structural characterization (e.g. via TEM, STEM, SEM, FIB-SEM/3D reconstructions).
At Topsoe, we’re constructing an SOEC manufacturing facility in Denmark, set to be operational in 2025. This facility will produce electrolysis cells, stacks and modules with a capacity of 500 MW per year. We’ve already announced the first agreement, a reservation of 500 MW capacity. However, in parallel with the up-scaling and commercialization of our TOPSOE™ SOEC electrolyzer technology, it is remains crucial to continue the R&D work on SOEC – cells, components, and stacks. In this perspective, this presentation will provide an overview of Topsoe’s expertise, results, and initiatives in the field of SOEC. This spans from studies of materials’ properties, electrode nanostructures as illustrated in Figure 1, to the electrochemical performance of cells and stacks, and the design of MW-sized systems. We’ll present examples of performance and durability testing and will concluded with a status on Topsoe’s up-scaling of SOEC production capacity.
Figure 1: Example of electrode nano-structure characterization via TEM of Ni/YSZ based fuel electrode produced and tested at Topsoe [1].
Gielgud-I1
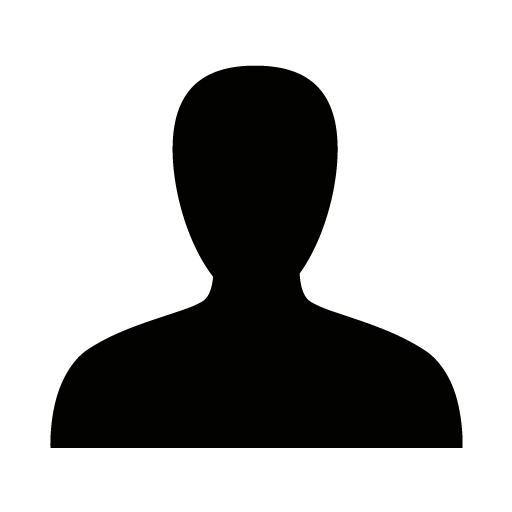
Over the past 10 years, significant development on materials, cells, stacks and sytem integrating proton-conducting oxides have been conducted in a series of European projects (WINNER, GAMER, PROTOSTACK). Proton conducting oxide materials have several unique characteristics that distinguish them from oxygen ion conducting oxides and proton-conducting polymers. By enabling proton-mediated electrochemistry under both dry and wet environments at moderate temperatures (e.g. 400–600 °C), these materials provide unique opportunities to enhance a diverse range of complementary electrochemical and thermochemical processes while providing storage solutions. Leveraging these characteristics, the projects have focused on designing, manufacturing, assembling and testing tubular cells and stacks to alleviate thermo-mechanical constraints when operating in high pressure (10 bar). As starting point, the cells contain BZCY-based electrolyte, BZCY-Ni tubular hydrogen electrode and BGLC-BZCY composite steam + O2 electrode. Further improvement of the cells is achieved by designing a dual layered electrode with addition of a BGLC top layer, as will be shown by comparing the electrochemical performance of these architectures. The cells are integrated in tubular steel shells forming the so called "single engineering units" (SEU). The SEUs have ca. 50-60 cm2 active surface area and are tested in pressurized steam electrolysis operation at intermediate temperature (600°C) and up to 10 bar pressure demonstrating high faradaic efficiency (> 80%) and reasonable area specific resistance. A dedicated design assembly of the SEUs to produce racks of 16 SEUs mounted in series has been developed in the project, as well as an integrated system design with necessary balance of plant components. Results of this system operated at ambient pressure and up to 7 bar will be presented. Knowledge gained from operations of the SEUs and system have highlighted the dramatic impact of Cr evaporation on cells performance, microstructure and composition, as will be presented. Mitigations strategies will also be discussed.
Gielgud-O1
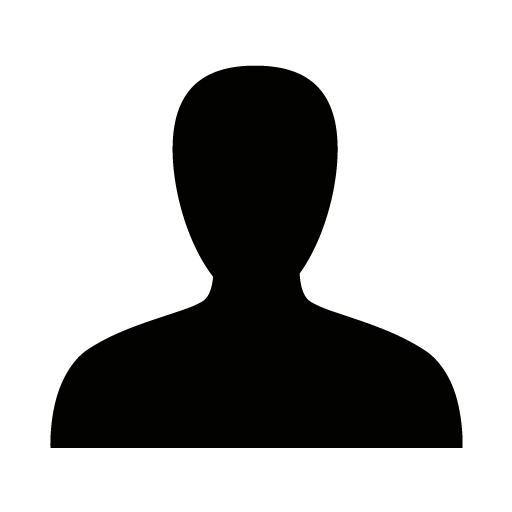
March 2011: Doctor Degree, Graduate School of Human and Environmental Studies, Kyoto University, Japan
April 2011: Joined Osaka Gas Co., Ltd (micro-CHP Development Department)
April 2012: Residential Energy System Development Department, Osaka Gas
July 2013: Residential Energy System Development Department, Osaka Gas (Assistant Manager)
April 2020: Energy Technology Laboratories, Osaka Gas (Chief Reseacher)
Solid oxide fuel cell (SOFC) and Solid oxide electrolysis cell (SOEC) stacks are assembly of metal interconnector, ceramic cells, and have multiple layers of various ceramics such as an electrolyte and electrodes. It is necessary to clarify the deterioration mode of each material and between materials to suppress the deterioration rate. Cr poisoning of the cathode was one of the most significant deteriorations in SOFC. As a candidate for suppressing Cr poisoning deterioration of cathodes, we have developed a ceramic coating for metal-interconnectors using an electrodeposition coating method and confirmed that it can suppress Cr poisoning under SOFC operating environments. SOFC cells, which consist of the electrolyte and electrodes, undergo large amount of deterioration, and many reports have been published on the reaction mechanism at the solid-solid interface and the deterioration due to impurity poisoning. However, in SOFC cell stacking, it is necessary to discuss the effects of ceramic interfaces on durability other than electrode reactions. For example, thermal cycles associated with startup and shutdown may cause peeling of multilayers interfaces and effects on other layers of ceramic bonding material such as bonding materials that electrically connect metal-interconnectors and SOFC cathodes. In this study, we have evaluated the effect on the long-term durability of the metal-interconnector / ceramic coating / bonding material multilayer interface to clarify the effect on the durability of SOFC and SOEC cell stacks. For the electrode contact materials, we selected the similar composition of Co-Mn spinel oxides as the electrode contact material so that it can be sintered even at lower temperatures than the general stacking temperatures. In designing the SOFC/SOEC interface, we focused on two methods: diffusion bonding and metal addition. The compositions of Co and Mn were graded at the ceramic coating / the electrode contact material interface. As a result, during stacking and during SOFC/SOEC operation, mutual diffusion occurred by virtue of the difference in activity between Co and Mn, and the ASR was reduced by improved adhesion between the ceramic coating and the electrode contact material. Mutual diffusion between the ceramic coating and the electrode contact material occurred over time during the long-term durability test, and the ASR continued to decrease. As a second method, by adding metallic Co to the electrode contact material (Co-Mn oxide) and sintered it, the adhesion at the ceramic coating/the electrode contact material interface was significantly improved. By utilizing the heat of oxidation when the metallic Co oxidized for sintering, we have achieved sintering at low temperatures by locally increasing the temperatures at the interface, even at low stacking temperatures. In the presentation, we will explain the design of the electrode contact material for SOFC cathodes and SOEC anodes and metal interconnectors based on the results of long-term high temperature accelerated tests more than 10,000 hours and the thermal cycle tests.
Gielgud-O2
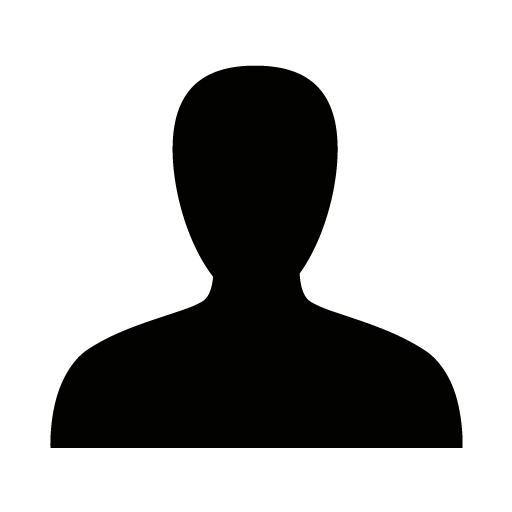
Proton Ceramic Electrolysis (PCEs) presents an intriguing advantage compared to other electrolysis technologies due to its potential for direct production of pressurized dry hydrogen, in addition to an intermediate operating temperature that allows for high electrical efficiency, thermal integration with industrial processes and renewable energy sources and slower diffusion-related degradation. Recent years have shown an impressive development in reported cell performances for PCEs, confirming the potential of the technology. Pressurized operation of PCEs is particularly advantageous due to a combination of favourable thermodynamics for proton transport in the electrolyte and the possibility of direct production of dry pressurized hydrogen without any downstream separation or compression.
This contribution will present the development and upscaling of tubular PCEs (10-60 cm2) based on a robust and chemically stable BZCY81 electrolyte for operation at high steam pressures. Scalable and reproducible production of tubular cells with a demonstrated tolerance to long-term operation at 10 bar operating pressure is achieved for more than 10 tubular cells with different cell configurations - highlighting the robustness of the proposed technology. Significant improvements in electrochemical performance and faradaic efficiency is achieved by optimizing the architecture and composition of the steam electrode. The impact of operating pressure on electrochemical activity, faradaic efficiency and overall energy efficiency is investigated at a single-cell level and discussed in terms of the defect chemistry of the electrolyte and the electrochemical processes at the electrode interfaces. Moreover, a combined electrochemical and fluid-dynamic model is used to corroborate experimental measurements over a range of process conditions to elucidate the impact of pressurized operation on the overall efficiency of PCEs, and discuss the trade-offs in different operating modes in terms of overall energy efficiency vs hydrogen production rate at a given delivery pressure at system level.
Gielgud-O3
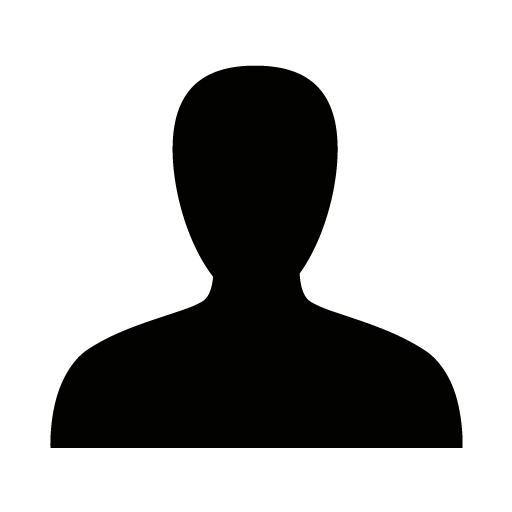
Electrochemical membrane reactors offer the opportunity to increase the yield of relevant chemical reactions such as the production of synthetic liquid fuels as well as to improve their energy efficiency by coupling exothermic and endothermic processes.
This work, developed in the frame of the EU Horizon 2020 “eCOCO2” project, presents the CO2 electro-catalytic reduction into methane by using tubular protonic membrane reactors. Two different modes of operation were employed to provide the needed H2 for the methanation reaction: H2 pumping and electrolysis. In both operation modes, the protonic membrane was composed of BaZr0.8Ce0.1Y0.1O3 as electrolyte and the cermet Ni+BaZr0.7Ce0.2Y0.1O3 as inner metallic electrode acting as support and as methanation catalyst. In the case of the H2 pumping, the outer electrode was made of Ni+BaZr0.7Ce0.2Y0.1O3 whereas for the electrolysis, an electrode made of PrBa0.5Sr0.5Co1.5Fe0.5O5+δ (PBSCF) was used.
Transport properties of the electrochemical cells were evaluated by impedance spectroscopy, i-V curves and H2 production as a function of the applied current density and the operational conditions, from 600 ºC to 450 ºC and pressures up to 30 bar. Then, methanation reaction performance was also evaluated as a function of the operational parameters, analyzing the CO2 conversion and CO and CH4 yields.
In both operational modes, it was observed that the total system pressure plays a key role in both the electrochemical and catalytic performances of the protonic cells. The improvement in the performance at high pressure is due to two effects occurring simultaneously as it was inferred from DRT analysis: (i) an increased hydration of the electrolyte which enhances the proton conductivity and (ii) the improvement of the surface kinetics and mass transfer of the electrodes. Regarding catalytic performance, CO2 conversion and CH4 selectivity increase significantly with pressure, reaching values of 86% and 94% respectively, for a stoichiometric H2/CO2 ratio of 4 at 450 °C and 30 bar.
In addition, computational fluid dynamics (CFD) simulations were conducted to gain critical insights into the reaction kinetics, transport phenomena, and electrochemical performance of the membrane reactor.
Fleming-K1
Ainara is a Tenured Scientist at the Intituto de Ciencia de Materiales de Madrid, CSIC and Visiting Reader in Energy Materials in the Department of Materials, Imperial College London. Her research focuses on the quantitative anlysis and optimisation of ion and electron dynamics in complex oxides, bulk surfaces and interfaces. She uses a combination of structural, chemical and electrochemical analysis including surface sensitive techniques and operando characterisation to develop the next generation of solid-state electrochemical devices such as metal anode all-solid-state batteries, low and intermediate temperature solid oxide fuel cells and electrolysers. She has been awarded with fellowships and grants as PI up to €3,3M and is involved in several UK , Spanish and European Commission projects. She has published over 80 (>3.2k citations h=29, i10=52) research papers in this field and holds 2 patents on their applications.
The development of all-solid-state batteries (ASSBs) has the potential to be transformational as a safer more efficient storage of energy for stationary and transport applications.
However, progress in developing well-performing and long-lasting ASSB cells has been delayed due to the difficulty to develop solid electrolytes that possess high cation conductivity combined with the adequate electro-chemo-mechanic stability required at cell level. This is associated to the complex and dynamic solid/solid interfaces that are generated during processing and that evolve upon cycling, which are difficult to study and therefore, optimize.
Most studies are trying to find a solution to this in the use of composite and hybrid solid electrolytes which adds even more complexity to understand which are the key factors governing the performance of the devices.
In this work, we analyze a range of inorganic and polymer-inorganic solid electrolytes with a combination of experimental and computational techniques, we discuss structural and chemical factors affecting the ion mobility in these systems and how it can be altered by the processing conditions at a bulk and interface level. High frequency (up to 3GHz) electrochemical testing allows for the quantification of ionic motion and separate bulk processes from processes taking places at the different interfaces. Synchrotron and neutron diffraction analysis is employed to understand how structural features and degrees of order-disorder might impact the cations conduction paths and conductivity. Surface-sensitive analysis ex-situ and operando are used to understand how the interfaces evolved and how this can be correlated to the electrochemical performance (1,2). Finally, good adhesion and controlled reactivity among materials is analyzed and routes for optimization are proposed.
Fleming-O1
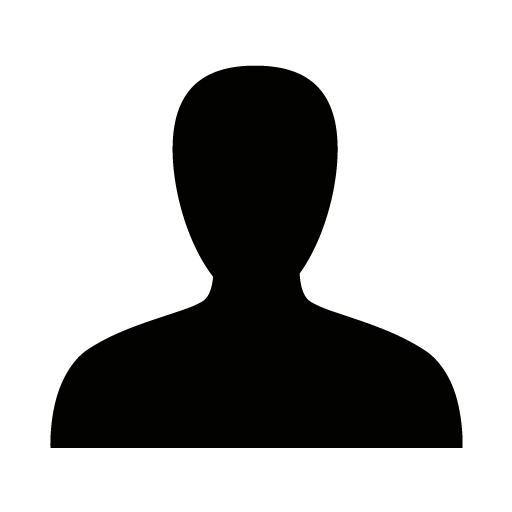
Thiophosphate solid electrolytes (SE) are promising candidates for solid state battery systems as they exhibit both reasonable ionic conductivity and ease of processability. Unfortunately, several challenges still hinder their commercialisation, one of them is linked to the control of the interfaces, and another one is linked to their electro-chemo-mechanical degradation. For the former, coating techniques can play a role to better handle the interfaces, whereas the second one is still unclear as it is declared that those degradation are electrochemical driven, when they could be there during the cell assembly, during the densification for example. Indeed, densification is a key parameter to control since it impacts the ionic conductivity, and ensures the contact between the active materials and the electrolyte1. Finally, proper densification of the SE could limit the propagation of dendrites. Investigating the densification is far from trivial, and here we rely on novel advanced in situ synchrotron techniques to provide new insight into the sintering of the electrolyte.
The densification of two thiophosphate electrolytes (one amorphous and one crystalline) was investigated using the UToPEc set-up at Psiché beamline of Soleil synchrotron. This unique set-up2 allowed for the simultaneous acquisition of absorption X-ray tomography, X-ray diffraction, density measurement and impedance measurements whilst applying a compressive force of up to 4 GPa meaning we can follow both structural and morphological changes during densification. As can be seen in Figure 1, the amorphous solid electrolyte can lose up to 53% of its own volume during the densification whereas the crystalline one could only be reduced by 20%.
Following the densification, we cycle the solid electrolyte to track the electro-chemo-degradation using X-ray diffraction computed tomography (XRD-CT) carried out at ID15a at the European Synchrotron Radiation Facility. It allows localizing the extend of the electrochemical and chemical degradation after long-term cycling and reveal that the thiophosphate solid electrolyte (LPSCL) decomposes electrochemically in known products such as S, Li2S, LiCl3 and that the chemical decomposition at the Li interface is more prominent than the electrochemical one.
Both advanced techniques reveal the difficulties in disentangling the extent of processing in solid state batteries.
Fleming-O2
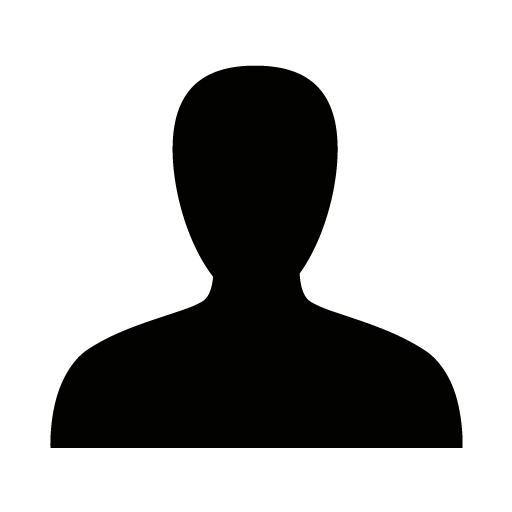
Li2S-P2S5 (LPS) glass-ceramic solid-state electrolytes (SSEs) have garnered substantial interest in solid-state battery research due to remarkable electrochemical properties and enhanced safety over conventional organic liquid electrolytes.[1, 2] While conventional mechanical synthesis techniques for processing LPS SSEs are generally energy-intensive and time-consuming, recent advances in solution processing techniques have produced LPS SSEs with exceptional ionic conductivities and mechanical properties at low reaction temperatures and in a fraction of the amount of time required by mechanical processes.[3] A major challenge lies in the limited-understanding of the underlying chemistries involved in solution processing, despite considerable efforts to elucidate full reaction mechanisms.[4] Here we contribute to the growing body of research investigating solvent and precursor interactions by exploring how the thiol functional group of the solvent ethyl mercaptan (EthSH) interacts with Li2S and P2S5 under common reaction conditions to facilitate the formation of LPS SSEs.
Three common molar stoichiometries of LPS systems (50:50, 70:30, 75:25) and the individual precursors Li2S and P2S5 were reacted in EthSH separately at room temperature for 24 hr with magnetic stirring, dried, and then annealed at 225 °C for 1 hr following common solution processing methodology.[5] During each process step, subsamples of each reaction were collected and characterized electrochemically and physiochemically by AC electrical impedance (EIS), powder X-ray diffraction (PXRD), Raman shift, and nuclear magnetic resonance (NMR) spectroscopies, and scanning electron microscopy.
The low boiling point of ethyl mercaptan allows for rapid drying of the processed SSEs. However, the solvent does not complex with Li2S and P2S5 when the Li2S content of the LPS system is at or above 70 mol%, as other polar organic solvents do, nor does it complex with the individual precursors. Instead, the 70:30 and 75:25 samples exhibited a moderate amount amorphous characteristic, commonly associated with solid-state reactions, and expressed XRD peaks assigned to Li2S and Li4P2S6. At 50 mol% Li2S, the ethyl mercaptan stabilized a Li2S-P2S5/EthSH complex similar to Li2P2S6 with a high order of crystallinity at room temperature, which was verified with PXRD, Raman, and SEM. All three systems exhibited low or immeasurable ionic conductivity with EIS. To the best of our knowledge room temperature crystallization of any LPS system has not been reported before.
Fleming-O3
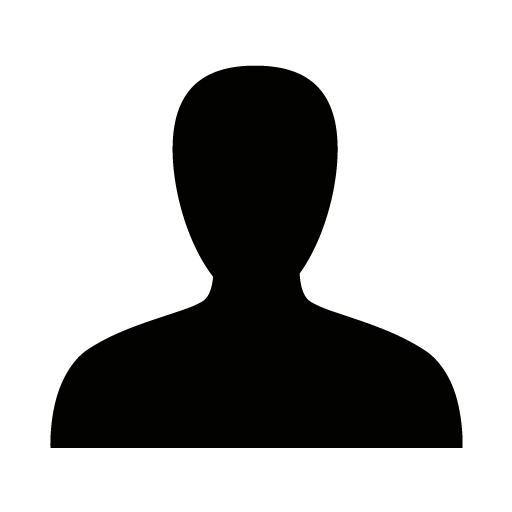
The all-solid-state battery (ASSB) is composed of a solid electrolyte, cathode and anode, unlike LIBs (lithium ion batteries) containing a combustible liquid electrolyte, and has recently been in the spotlight for its advantages of high energy density as well as high safety.1 A sulfide-based all-solid-state battery with a high ion conductivity (10-3 to 10-2 S cm-1) of solid electrolytes is attracting particular attention as a high-capacity and high-power battery.2 The sulfide-based solid electrolyte, the core material of this battery, has the advantage of being stable against heat, so when designing battery modules and packages, it is expected to increase the energy density at the package level by simplifying the cooling parts.
The sulfide-based solid electrolyte has high reactivity with atmospheric moisture and generates hydrogen sulfide (H2S), which leads to an increase in process cost and safety issues when mass-producing the battery. In order to overcome this, it is necessary to improve the moisture stability of the solid electrolyte. Through many studies, it has been confirmed that moisture stability is able to be improved through substitution or doping of oxides3, however the conductivity is largely reduced, and further improvement is required. In this study, as a way to improve the problems, we combined Argyrodite solid electrolyte and ion conductive oxide (Li3PO4) to minimize the decrease in ionic conductivity and improve atmospheric stability. In addition, the electrochemical stability of the material was investigated by evaluating the electrochemical properties to which the high-capacity cathode material (NCM811) was applied as pouch-type all solid state battery cell.
Fleming-O4
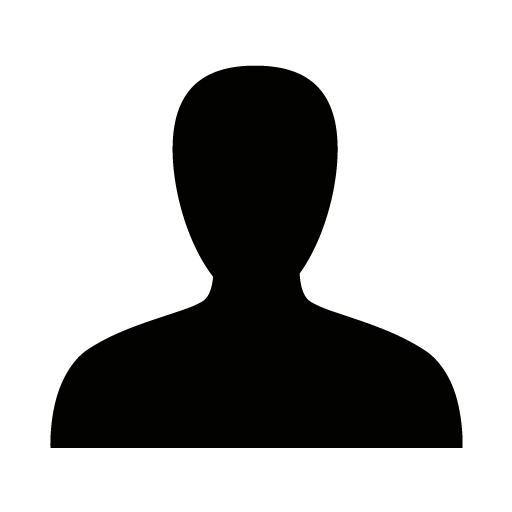
All-solid-state batteries with non-flammable inorganic solid electrolytes are a key technology to address the safety issues of lithium-ion batteries with flammable organic liquid electrolytes. However, conventional electrode materials suffer from substantial volume change during lithium-ion (de)intercalation, leading to the failure of the interface between the electrode materials and solid electrolytes and then severe performance degradation. In this work, we report strain-free charge storage via an interface between a transition-metal carbide nanosheet (MXene) and solid electrolytes, where MXene shows negligible structural change during lithium-ion (de)intercalation. Combined assessment including operando STEM-EELS elemental mapping clarified the strain-free nature of the MXene electrodes in the all solid-state batteries. In addition, the irreversible reactions at the MXene-electrolyte interface is visualized, explaining the inital irreversible capacities and relatively low rate capability of the MXene electrodes. A strain-free all-solid-state battery, which consists of Ti3C2Tx anode and disordered rocksalt Li8/7Ti2/7V4/7O2 cathode, demonstrates a long-term operation owing to the strain-free nature of both electrode materials.
Westminster-K1
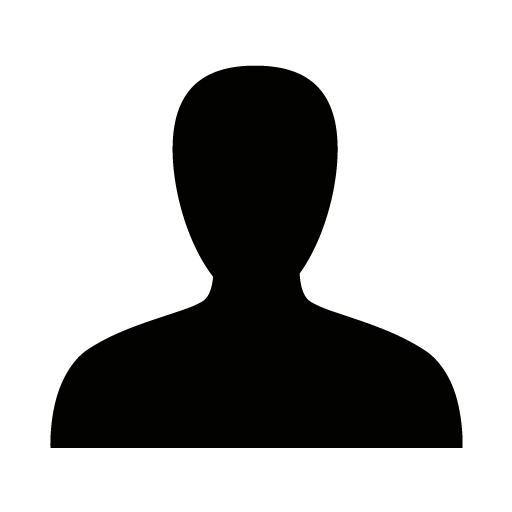
Dr Isaac Abrahams is Head of the Department of Chemistry at Queen Mary University of London and is a former Chair of the Royal Society of Chemistry Solid State Chemistry Group. His main area of research can broadly be defined as solid state and structural chemistry with close to 250 papers published in this area. Much of his work has been in the field of energy materials, focussing on ionically conducting solids for application as electrolytes in batteries, fuel cells and gas sensors, as well as ferroelectric materials for energy storge devices. In addition to the synthesis of new materials, he has developed expertise in defect structure determination using neutron and X-ray powder diffraction techniques and in conjunction with a.c. impedance spectroscopy studies has used this to examine structure-conductivity relationships and conductivity mechanisms in a variety of materials. This work has been aided by the development of total X-ray and neutron scattering methods, where both long-range and short-range ordering can be characterized simultaneously using a Reverse Monte Carlo approach to modelling the scattering data. Although much of his work has been on crystalline solids, Dr Abrahams has made use of his background in structural inorganic chemistry in the study of amorphous solids for applications such as all-solid-state batteries and biomedical materials. Here diffraction techniques are of limited use and a variety of other structural probes such as neutron scattering, solid-state NMR spectroscopy and EXAFS are required.
Fast oxide-ion conducting solids are used as electrolytes in solid oxide fuel cells (SOFCs), solid oxide electrolyser cells (SOECs), gas sensors, and oxygen pumps. Commercial devices are typically based on electrolytes showing a defect fluorite structure. The fluorite structure is well-known as a host for fast oxide-ion conduction, with archetypal examples including yttrium doped zirconia, delta-Bi2O3 and lanthanide doped ceria. High oxide-ion conductivity in these systems is facilitated by large concentrations of oxide-ion vacancies within the cubic fluorite structure. While the long-range structures of these materials are similar and essentially based on a cubic close packed array of cations with anions in the tetrahedral sites, local structure can vary significantly, particularly with respect to dopant cations and oxide-ion vacancies.
Until fairly recently, analysis of local structure in such systems relied on careful examination of average structural models, derived from diffraction data, to speculate on local coordination environments, in some cases supported by local structural probes such as solid-state NMR and EXAFS as well as computational modelling. However, recent developments in reverse Monte Carlo (RMC) analysis of total neutron scattering data have allowed for a more detailed analysis of local structure based on physical data using a combination of Bragg and diffuse scattering. The former is associated with the long-range order while the latter arises from short-range order. The large box approach of RMC modelling uniquely allows for examination of the resulting model for local vacancy ordering as well as preferred vacancy association and dopant clustering.
Using this approach we have examined local structure in a number of fluorite structured systems. In the case of delta-Bi2O3, the highly conducting fluorite phase is only stable above ca. 730 °C but can be preserved to room temperature through incorporation of dopant cations. However, even for isovalent dopants, where the nominal vacancy concentration is unaltered through doping, conductivity is lower than in the parent delta-Bi2O3, and has been associated with oxide-ion vacancy trapping by the dopant cation. In early work, using RMC modelling of total neutron scattering data we were able to show that local ordering of oxide-ion vacancy pairs occurs in these systems with a statistically non-random preference for ordering in the <100> direction.1,2,3
In seeking to lower the operating temperature of SOFCs, electrolytes based on lanthanide doped cerias, such as gadolinium doped ceria (GDC), have been utilised. Substitution of tetravalent Ce4+ by trivalent lanthanide (Ln3+) ions (Ce1-xLnxO2-x/2) introduces oxide ion vacancies into the fluorite lattice. We have carried out RMC analysis of neutron total scattering data from Nd doped ceria and GDC. In the latter case, samples prepared with isotopically enriched 160Gd were used to overcome the high neutron absorption coefficient of naturally abundant Gd.
The study reveals three distinct features in the local structure of lanthanide doped cerias. Firstly, clustering of the dopant cations, secondly preferred association between oxide-ion vacancies and the dopant cations and finally oxide-ion vacancy clustering. Details of these findings will be presented.
Westminster-O1
For a sustainable energy future, solid-oxide fuel cells (SOFC) play an essential role: they generate electricity from industrial waste gases, with water as their only byproduct. The cornerstone of this technology are materials capable of splitting gaseous oxygen (O2) to conduct it as oxygen anions (O2-) in the device.1 Commercial SOFC currently rely on strontium-doped lanthanum perovskites (La1-xSrxMO3-d with M=Co, Mn, Fe) as a cathode. The high-performance of this perovskite comes from three key factors: the atomic arrangement is cubic, it is inherently oxygen deficient, and contains multivalent metals that allow for mixed ionic-electronic conductivity. However, the activation of the oxygen-ion conductivity requires high temperature (T > 800°C), and lanthanum is a rare-earth element with extreme supply issues.2,3
The quest for new materials with equivalent (or improved) performances and a more attractive elements composition starts with the need to stabilise a cubic perovskite arrangement, optimal for ionic and electronic conductivity. However, with the same ideal composition (ABO3, where A = non-metal, B = metal) the cubic structure might be unachievable or unstable.
The established approach in materials chemistry is to mix the components in B with one element at a time (A(B’B’’)O3).4
A well-known, rare-earth free material for fuel cells is BaInO2.5 5, whose ionic conductivity (normally active at temperatures above 1040°C) can be lowered with a variety of doping of the B-site (V, Mo, W, Cu, Ga, Y, Zr, Sn, Ti, Al, Fe, Co, Mn, etc) to yield a cubic perovskite 6-13. This compositional flexibility makes it a great candidate for a systematic study on the synthesis of high-entropy perovskites (HEPs): a cubic perovskite in which the B position is equally shared by five or more atoms, where the disorder of a multi-element system creates an entropic advantage. Once synthesised, HEPs are often reported to be better performing their they low-doping equivalent, with lower activation temperature and higer conductivity. 14
In this study we perform a systematic study on sequential on multi-elemental doping of BaInO2.5, attempting a rational incremental approach of 2->3->4->5 elements sharing the B position, taking from a pool of 10 elements (In, Sn, Ti, V, Cr, Mn, Fe, Co, Ni, Zr) that exclude rare-earth materials. All the syntheses were performed with solid state ceramic methods, with maximum reaction temperature fixed at 1100°C.
We will highlight 8 new stable compositions in the BaInxM1-xO3-d (M = mixed metal) compositional space, of which three medium entropy and one high entropy. The structural results in this system highlight a complex interplay between charge, size and orbital configuration and the capability (or not) to form a multi-doped system. The thermogravimetric characterisation provides insight on the protonic and electronic conductivity performance, in terms of amount of mobile vacancies and the temperature of activation for their mobility.
This systematic study opens up to answer the question: is high-entropy stabilisation always achievable, and always beneficial, for a functional material?
Westminster-O2
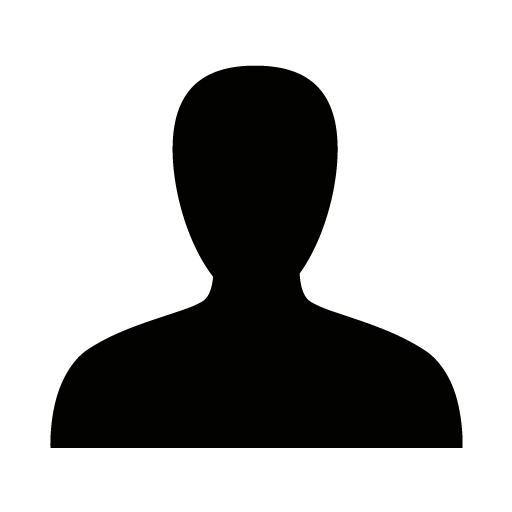
The drastic Effect of A-site non-stoichiometry on the Cation Diffusion and Core-Shell Formation in NBT-based Ceramics
Sophie Bauer a, Till Frömling a,b
a Technical University of Darmstadt, Department of Materials and Earth Science, Peter‑Grünberg‑Straße 2, 64287 Darmstadt, Germany, sophie.bauer@mr.tu-darmstadt.de
b Fraunhofer Research Institution for Materials Recycling and Resource Strategies (IWKS), Aschaffenburger Straße 121, 63457 Hanau, Germany
Since Bi2O3 tends to evaporate easily during the solid-state synthesis of Na0.5Bi0.5TiO3‑based (NBT-based) ceramics, it is extremely challenging to control the oxygen and bismuth vacancy formation, and consequently, the stoichiometry of the composition. [1] As the diffusion processes in NBT‑based ceramics strongly depend on the vacancy concentration, the addition of 0.1 % bismuth can supress the grain boundary diffusion coefficient by six orders of magnitude. [1] Therefore, different microstructures, each with distinct properties, emerge depending on the degree of bismuth deficiency and, consequently, the concentration of oxygen and bismuth vacancies. [1, 2] In the case of Na0.5Bi0.5TiO3‑SrTiO3 (NBT-ST), a homogeneous material can be synthesized by introducing a slight bismuth deficiency in the starting material, while bismuth enrichment results in a core-shell microstructure. [2, 3] As expected, both materials exhibit fundamentally different properties. Bi-enrichment causes an exceptionally high achievable strain in this ferroelectric material and a high energy density that can be stored, offering potential applications in actuators and high-energy capacitors. In contrast, Bi deficiency results in a seven times larger grain size and significantly higher conductivity.
This work sheds light on the possibilities of tailoring the microstructure, transport properties, and electromechanical properties of NBT-based materials. Understanding the diffusion mechanisms of cations in NBT-based ceramics is inevitably crucial in this context.
Westminster-O3
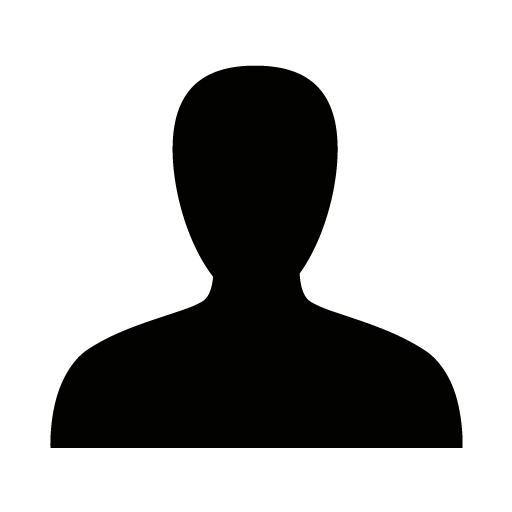
Photovoltaic (PV) conversion, as an eco-friendly energy solution to contemporary challenges, has garnered extensive attention, particularly in studying PV cells at ambient temperatures. Notably, the renowned photocatalyst SrTiO3 has exhibited photo-responsiveness even at high temperatures [1], achieving a remarkable photovoltage exceeding 1.0 V [2] and a high short-circuit current density of approximately 1.5 mA•cm-2 [3] under optimized conditions.
Detailed analysis reveals that the induction of photo-response at high temperatures is influenced by photo-oxidation in SrTiO3 and the heterojunction between the photoelectrode and SrTiO3. Inspired by these insights, our research group explored the photo-response of ionic conducting materials at high temperatures. While experimenting with SrTiO3 deposited on Gd-doped CeO2 (GDC) electrolytes, it was found that the bare GDC electrolytes also showed a photo response under heating. This study focuses on the photo response of GDC at high temperatures and aims to unveil the photovoltage mechanism.
In our experiments, sintered GDC was mirror-polished, and Pt and Au electrodes were sputtered onto it. The samples were placed in a quartz chamber and heated within the range of 200 ºC to 400 ºC under O2-Ar gas flow. Open circuit voltage (OCV), electrochemical impedance spectroscopy (EIS), and DC polarization measurements were conducted under both UV (365 nm) irradiation and non-irradiation conditions. This presentation will discuss the impact of gas composition and temperature on the photo-response of GDC. Additionally, the contribution of oxygen transport on photovoltage will be validated by measuring the changes in oxygen partial pressure during UV irradiation using a yttria-doped zirconia oxygen sensor.
St.James-K1
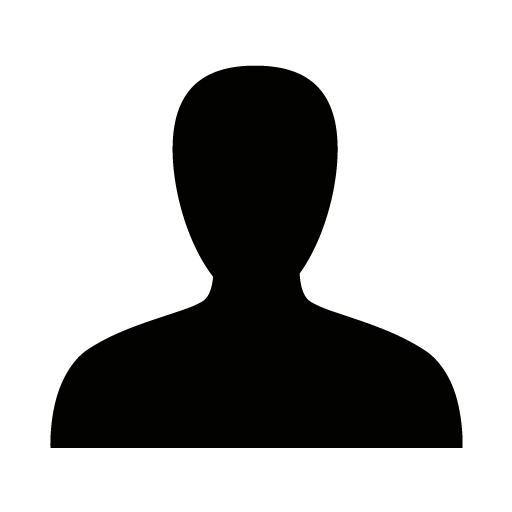
Ba(Zr,Ce,Y)O3-δ proton-conducting materials: manufacture, performance and stability
W. A. Meulenberg1,2,*, J. Malzbender1, J. L. Wolter1, W. Deibert1, O. Guillon1, J. M. Serra3,
C. Segarra3, , A. Dos Santos3, L. Almar3, D. Catalán-Martínez3, M. Fabuel3, S. Escolástico3,
W. Zhou1
1 Forschungszentrum Jülich GmbH, Institute of Energy and Climate Research (IEK), 52425 Jülich, Germany
2 University of Twente, Faculty of Science and Technology, Inorganic Membranes, P.O. Box 217, 7500 AE, Enschede, the Netherlands
3 Instituto de Tecnología Química, Universitat Politècnica de València – Consejo Superior de Investigaciones Científicas, Av. Los Naranjos, s/n, 46022 Valencia, Spain
Abstract
Proton-conducting membranes have great potential for applications in proton conducting membrane reactors for the production of commodity chemicals or synthetic fuels as well as for use in solid oxide fuel cells. Ba(Zr,Ce, Y)O3-δ perovskite oxides are of particular interest due to its proven high proton conductivity and good chemical stability. In this work, fabrication of Ba(Zr,Ce, Y)O3-δ samples, including thick pellet, thin tape-cast and multi-layered half-cell, were studied to prevent the typical challenges like warping, cracking and delaminating. Materials with different stoichiometries were investigated, on their conductivity and chemical stability against NH3 and H2O, to select the most promised candidate for future compact membrane reactor. Mechanical behaviors including elastic modulus, hardness, fracture toughness and creep were investigated to warrant long-term structural stability. Thus, a systematic investigation on Ba(Zr,Ce, Y)O3-δ materials was carried out to pave the road towards widespread application of proton-conducting membranes.
St.James-O1
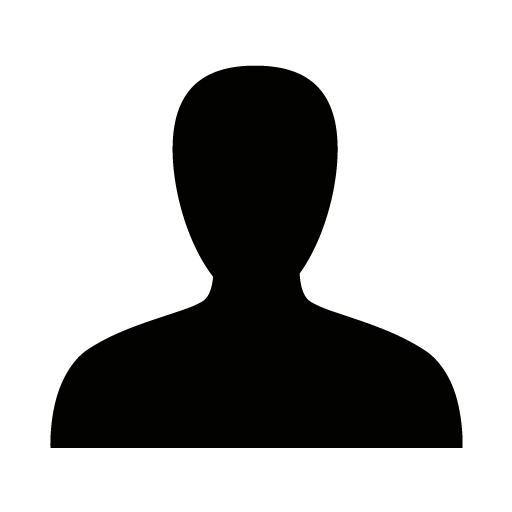
The synergy between ionic liquids (ILs) and metal-organic frameworks (MOFs) has attracted considerable scholarly attention as an innovative hybrid ionic conductor [1-2]. The incorporation of ionic liquids into the nanomaterial structure of MOFs has led to the successful synthesis of a novel solid electrolyte characterized by exceptional thermal stability and high ionic conductivity [3]. Here, we use an efficient capillary action method to introduce two proton conductive ionic liquids, namely 1-Ethyl-3-methylimidazolium triflate ([Emim][TfO]) and 1-Ethyl-3-methylimidazolium bis(trifluoromethylsulfonyl)imide ([Emim][TFSI]), into the pores of ZIF-8, separately. Our investigation focuses on the influence of varying ILs quantities on pore volume, thermal stability, microstructure, and proton conductivity. The cages of ZIF-8 expand, and the decomposition temperature decreases following the encapsulation of ILs molecules. Notably, when the pores of the ZIF-8 material are entirely saturated with [Emim][TFSI], the composite material exhibits an impressive ionic conductivity of 1.140 mS·cm−1 (at 0 % RH and 180 °C). The activation energy for this particular composite is 0.25(4) eV, which is notably low when compared to other IL@MOF conductive composites. These findings underscore the significant promise of IL@MOF hybrid composites as potential ionic conductors, attributable to their elevated electrical conductivity and relatively low activation energy. Additional, this composite can be used as electrolytes of High-Temperature Proton Exchange Membrane Fuel Cells, which are a type of fuel cell that operates at higher temperatures compared to traditional proton exchange membrane fuel cells (PEMFCs).
Literature:
[1] J. Jia et al, ACS Sustain. Chem. Eng. 2023, 11, 13502-13507. [2] A. B. Kanj et al, Nano Lett. 2019, 19, 2114-2120. [3] W. L. Xue et al, Angew. Chem. Int. Ed. Engl. 2021, 60, 1290-1297.
St.James-O2
Donor-substituted (W, Mo) LaNbO4 materials have been reported to be good oxide ion conductors exhibiting excellent chemical stability with acceptable ionic conductivity, while their protonic conductivity is negligible [1]-[6]. However, most of these works were conducted at ambient conditions, and the conductivity behaviours at reducing conditions can be significantly different from non-reducing conditions.
In this work, LaNb0.84W0.16O4+δ and LaNb0.84Mo0.16O4+δ have been successfully synthesized via a solid-state reaction route. An abnormal conductivity behaviour at reducing conditions was found in electrochemical impedance spectroscopy measurements. At first, this abnormality is attributed to the emergence of the electronic conductivity. However, more evidence from different characterisations such as DC measurement, XPS and XAS suggest the simultaneous emergence of protonic conductivity later on. Protons originating from the protonic incorporation are indeed participated in the conductivity behaviour and worth further investigating.
In conclusion, the conductivity behaviour can be significantly different depending on the dopants. For W-substituted LaNbO4, the materials are stable at reducing conditions without the change of oxidation states of cations as well as the oxygen stoichiometry. Therefore, the electronic conductivity in W-substituted LaNbO4 is surprisingly low, and the conductivity enhancement is mainly, if not all, from the emergence of the protonic conductivity. In contrast, Mo-substituted LaNbO4 materials suffer from severe reductions and their conductivity behaviour is more complex than W-substituted LaNbO4 as there is a trade-off between the emergence of the electronic and protonic conductivity and the degradation of oxide ionic conductivity.
St.James-O3
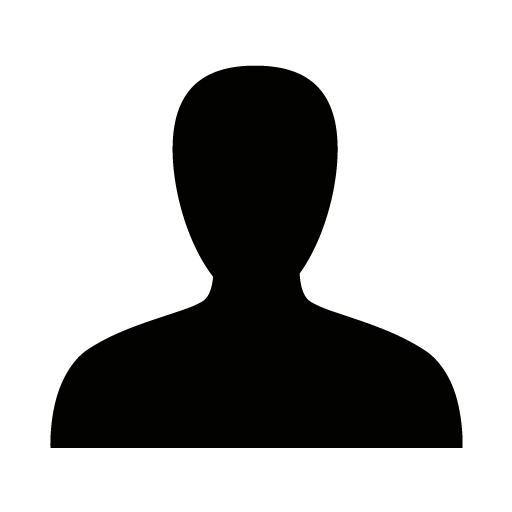
Discovering efficient and innovative materials for protonic ceramic cells requires the characterization of countless compositions. The knowledge of structural properties, hydration parameters, and electrochemical performances is essential for selecting the most promising electrolyte materials. However, traditional measurements are performed on individual samples and require extremely long stabilization times. A high-throughput approach was chosen to evaluate the electrochemical performance of hundreds of compositions inside the Ba(Ce,Sn,Zr)0.8Y0.1Yb0.1O3-δ (BCSZYY) ternary system. Thin film combinatorial libraries were produced by pulsed laser deposition on 100 mm diameter wafers. The composition gradient is obtained through alternate depositions of BaCe0.8Y0.1Yb0.1O3-δ (BCYY), BaSn0.8Y0.1Yb0.1O3-δ (BSYY), and BaZr0.8Y0.1Yb0.1O3-δ (BZYY) centered on three opposite edges of the substrate to recreate the ternary diagram. XY-resolved characterization techniques were performed to map the elemental, structural, and hydration properties of each composition inside the BCSZYY ternary system.
In particular, X-ray diffraction (XRD) was carried out to study the thin film structure. More than 300 diffractograms were collected on different positions of a single sample and analyzed through a custom-made code to calculate the pseudocubic cell parameter of the thin film. The resulting values of the "a" cell parameters range from 4.176 Å for the BSYY‑rich part, to 4.251 Å for the BZYY area, up to 4.418 Å for the BCYY one, in good accordance with the values obtained by Rietveld refinement for reference powders of single materials.
In situ measurements were performed at the DiffAbs beamline of the SOLEIL synchrotron by simultaneously collecting X-ray diffraction and fluorescence signals. XRF allowed the evaluation of the elemental distribution of the BCSZYY samples at room temperature and gave insight into thickness variation, complementary to spectroscopic ellipsometry results. A custom-made furnace was specifically developed to perform in situ XRD measurements on wide-surface thin-film samples at high temperatures in dry and wet conditions. The furnace consists of a hotplate and an X-ray transparent PEEK dome, allowing the control of temperature and atmosphere. Due to the large surface of the hotplate, the temperature distribution was calibrated using a platinum internal reference. The BCSZYY sample was subsequently measured in dry and wet N2 to calculate the unit cell expansion due to water incorporation and to extract some information about hydration thermodynamics for the entire BCSZYY ternary system.
A custom-made setup for electrochemical impedance spectroscopy measurements is under construction to measure the conductivity of the entire ternary system. Electrochemical results will be related to the diffusion coefficients obtained using 18O2, H218O, and D2O isotopic exchange followed by Time of Flight Secondary Ions Mass Spectrometry (ToF-SIMS). Recently, we successfully applied this technique to combinatorial samples, and it will be systematically performed inside high-throughput procedures.
Moore-K1
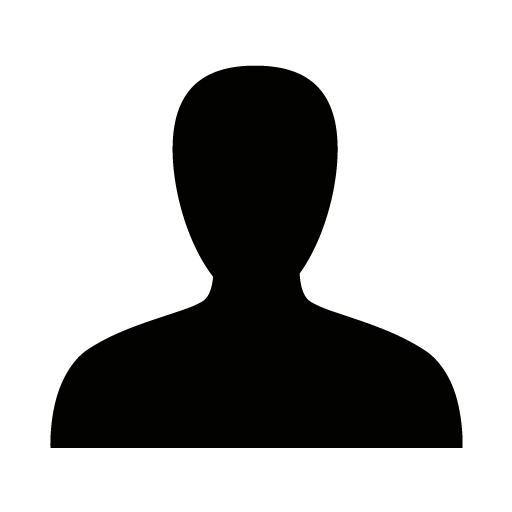
Electrochemical devices using a solid electrolyte are expected as next-generation energy conversion and storage devices. Typical examples are solid oxide cells using a solid-state oxide-ion (SOC) or proton conductor (PCC) and all-solid-state batteries using a solid-state lithium-ion conductor (ASSB). In such devices, the difference in chemical potential of reaction component, which is maintained across the electrolyte between the cathode and the anode, is the driving force for ionic transport and reaction. The chemical potential in the electrolyte also has a great impact on thermodynamic stability of the electrolyte, thus the device performance and durability [1, 2]. Therefore, in order to optimize designs and operating conditions of the devices or to ensure stability and durability of the devices, it is important to understand the chemical potential distribution in solid electrolyte under operation.
In this study, we aimed to experimentally evaluate the chemical potential distribution in solid state ionics devices. So far, a lot of numerical studies have been carried to estimate the chemical potential distribution in solid state ionics devices [1-3]. However, only a few studies have been reported to directly observe the distribution of chemical potential in an experimental manner [4-6]. And as far as the authors know, there exist no studies for its operando observation under device operation.
Challenges for the direct observation of chemical potential in solid state ionics devices are (i) how to detect the chemical potential change in the solid electrolyte and (ii) how to achieve operando measurement with high positional and temporal resolutions at desired temperature in controlled atmosphere under polarization. For the detection of chemical potential change, we embedded a potential probe into or on the electrolyte. In addition, as an operando analytical technique with high positional and temporal resolutions, micro-beam X-ray absorption fine structure (XAFS) was employed. In this presentation, our recent results on the direct observation of chemical potential distribution in SOC and ASSB will be given. The distribution of oxygen chemical potential in the former and that of lithium chemical potential in the latter, respectively, were evaluated under device operation. The obtained results were compared with the simulated results based on the ambipolar diffusion of ion and electron in the electrolyte.
Moore-O1
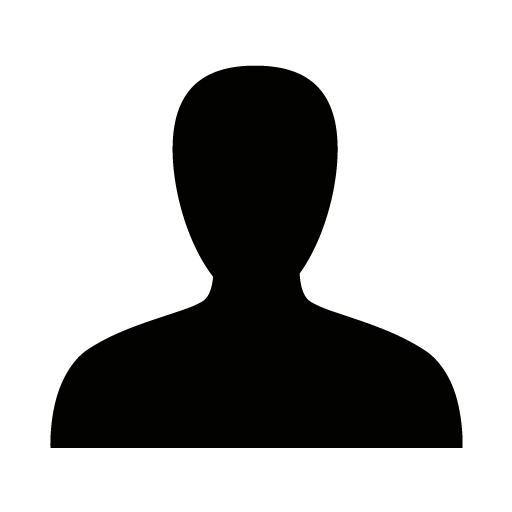
High-temperature electrolysis is a compelling choice for efficient renewable energy storage, with electrochemical CO2 and H2O splitting serving as key reactions. Solid Oxide Electrolysis Cells (SOECs), known for their stability at elevated temperatures, stand out for such applications. Perovskite-type and fluorite-type oxides functioning as Mixed Ionic and Electronic Conductors (MIECs) prove to be well-suited materials for SOEC cathodes. Crucial properties for these materials include catalytic activity, electronic and ionic conductivity, redox-cycling stability, and resilience against graphitic carbon deposition during CO2 splitting. However, many available materials combine only some of these properties, or degrade in the course of operation.
In order to enhance the efficiency and stability of SOEC cathode materials, it is imperative to attain a fundamental understanding regarding the mechanisms of degradation processes and surface reactions. This objective can be achieved through the utilization of in-situ surface-sensitive measurements, such as in-situ X-ray Photoelectron Spectroscopy (XPS) or in-situ Scanning Electron Microscopy (SEM). By combining these techniques with precisely crafted model electrodes, fabricated using Pulsed Laser Deposition (PLD) and magnetron sputtering, it becomes possible to correlate the surface reaction rate to surface compositions and morphologies.
Particularly intriguing materials for CO2 and H2O splitting are metal/metal-oxide composites. The interactions of the metal with both the supporting oxide and the gas phase significantly influences the surface activity of cathode materials in high-temperature electrolysis. Examples include B-site exsolved nanoparticles on perovskite-type oxides as well as the dewetting behavior of metallic nickel on Gd-doped Ceria (GDC). In both cases, differences in atmosphere, temperature and applied voltage lead to surface chemical and morphological changes, which furthermore influence the surface activity as well as the formation kinetics of undesired byproducts like graphitic carbon in the case of electrochemical CO2 splitting.
In conclusion, our study on metal-support interactions on SOEC cathode materials using in-situ surface-sensitive measurements provides valuable insights for enhancing the efficiency and stability of high-temperature electrolysis for renewable energy storage.
Moore-O2
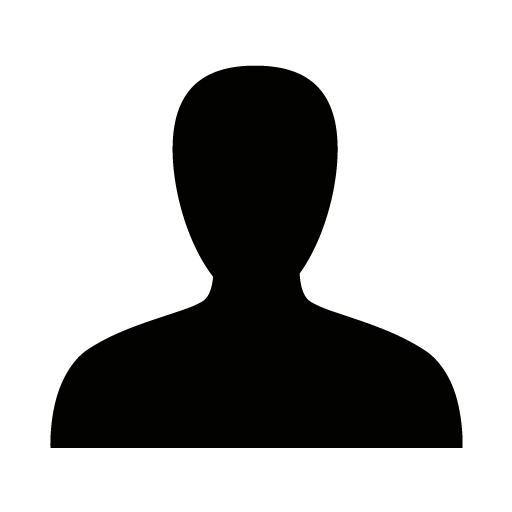
Mixed ionic electronic conductors (MIECs) are an important class of materials characterized by simultaneous conduction of ions and electrons. One key aspect of this family of materials is the ability to vary their concentration of charged point defects (e.g. vacancies, interstitials, substitutional ions) through electrochemical ionic insertion. Indeed, the application of an electrochemical potential across an electrolyte can modify the chemical equilibrium of point defects in MIEC materials, forcing a variation of the defect’s concentration. This fundamental characteristic has allowed the development of non-stoichiometric materials as efficient insertion electrodes for Lithium-ion batteries or solid oxide cells’ electrodes for hydrogen technology. Additionally, the strong correlation between ionic point defects and structural/electronic properties offers a useful approach to modulate their functional properties, such as magnetism for novel magnetoionic devices. For all these systems, it is of high importance to understand equilibrium and kinetics of ionic insertion, possibly in-situ and/or operando.
In this work, we present optoionic impedance spectroscopy (OIS) as a novel technique to study ionic insertion in MIEC thin films. OIS is a direct modification of Electrochemical Impedance Spectroscopy (EIS), one of the most common techniques employed in the study of electrochemical systems. Indeed, both techniques involves the application of an external sinusoidal electrical stimulus able to promote ionic insertion over a wide range of frequencies. However, while EIS measures the sinusoidal electrical response of the system, OIS focuses on the dynamic optical properties. Thanks to the great sensitivity of optical properties of many MIECs to the concentration of point defects, ionic insertion can be studied dynamically.[1] In contrast with previous works,[2] we employed spectroscopy ellipsometry for increasing the sensitivity of OIS, a technique able to optically detect various structural and optical attributes of a thin film, including optical constants and thickness, regardless of the substrate's optical properties.
Different case studies were devised for the demonstration of OIS technique capabilities. First, we investigated the OIS response to oxygen insertion in La0.5Sr0.5FeO3−δ (LSF50) thin films deposited on Yttria-stabilized Zirconia electrolyte. By comparing EIS and OIS we demonstrated that while the former measures the variation of charge in time (i.e. current) of the system, the latter probes the variation of chemical charge in the layer (i.e. variation of charged ions). Moreover, we showed that, as long as stimuli-response linearity is maintained, OIS can be used to retrieve the chemical capacitance without employing an ellipsometry optical fitting model. Then, we applied OIS for the characterization of the oxygen insertion in SrFeO3−δ (SFO) thin films, a material characterized by a complex topotactic phase transition under reducing conditions. Studying the OIS response as a function of different equilibrium potential, we demonstrated that OIS technique can be employed to obtain the concentration of oxygen in a material with a complex defect chemistry such as SFO. Finally, we showed that OIS can be also employed for study the kinetics of ion insertion in LSF50 thin films in liquid alkaline electrolyte, where a significant contribution from ion diffusivity is observed. Overall, OIS is presented as a powerful technique for studying equilibrium and kinetics of ionic insertion in MIEC thin films.
Moore-O3
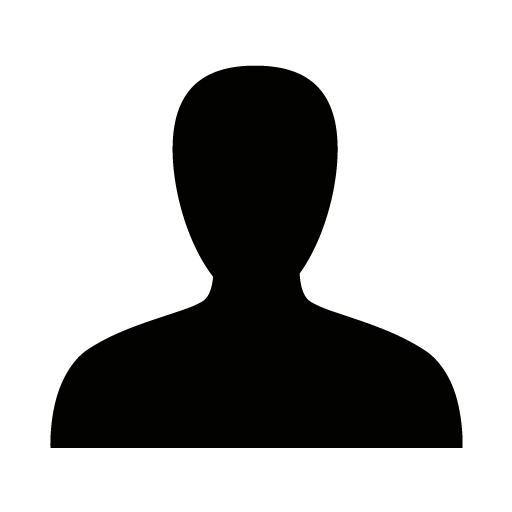
The composite of nickel and yttria stabilised zirconia (Ni-YSZ) is a frequent choice as the fuel electrode in solid oxide cells (SOC). Despite progress in electrode research, many degradation mechanisms are still up for debate and degradation is usually correlated with operating conditions. However, the determination of degradation mechanisms during device-scale testing remains complicated due to the intertwined effects of temperature, gas phase, overpotential and material properties. The microstructural variations in the porous electrodes introduce further uncertainties as local conditions vary and destructive sample preparation is required to assess the microstructure. This makes it difficult to directly relate microstructural changes to the electrochemical measurements.
Hence, complementary tools and experiments are warranted. Here, we present an approach based on a thin nickel film/YSZ electrode featuring a well-defined and controlled triple phase boundary for the conversion of H2O to H2 or vice versa without distributions in temperature and gas phase. Sample fabrication in clean room facilities using photolithography and magnetron sputtering provides micrometre-controlled electrode dimensions. Single atmosphere testing with a non-polarised control probe electrode grants the opportunity to separate potential induced degradation from temperature and gas phase driven effects.
During durability tests, the impedance response is measured while the phase stability and defect concentration is monitored by operando micro-Raman spectroscopy. The restructuring of nickel is tracked by ex-situ atomic force microscopy and electron microscopy, whereas x-ray photoelectron spectroscopy and energy dispersive x-ray spectroscopy are used to analyse material compositions. In addition, alloying of nickel and co-doping of YSZ are investigated for a possible suppression of degradation and to improve the mechanistic understanding. Results on nickel mobility, impurity effects, and interfacial contact loss are presented for Ni/YSZ, alloyed Ni, and co-doped YSZ.
Moore-O4
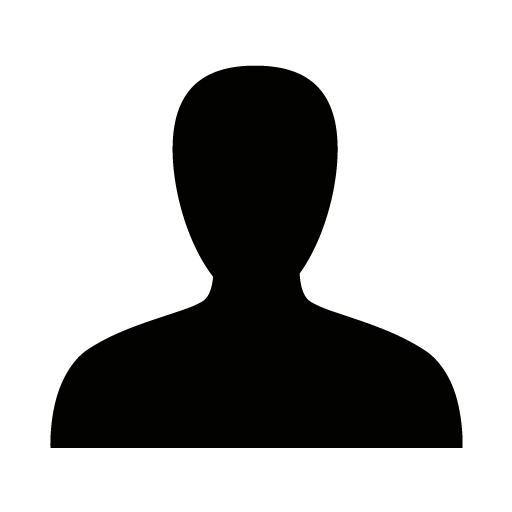
Transition metal doped SrTiO3 is of significance for devices in energy1,2, information3,4, and catalysis5. With the growth characterization of Ni-doped SrTiO3 (Ni:STO), spontaneous formation of separated nano-phases is observed. In view of controlling the formation of such nano-phases and in a wider context for vertically aligned nanocolumn engineering6, our focus is on the growth mechanism of Ni:STO with Ni dopant enrichment in designated areas of the perovskite lattice, associated to the occasional formation of nano-phases embedded in a Ni-doped STO matrix.
Scanning Tunneling Microscopy and Spectroscopy (STM/S) are unique surface techniques for mapping both precise topography and electronic properties down to the atom scale, making them a promising instrument for studying nanostructures, even within device structures7.
In this study, we investigate the reflection high-energy electron diffraction-controlled pulsed laser deposition (PLD) grown 0.25 unit cell (u.c.), 0.5 u.c., and 10 u.c. Ni:STO on Nb-doped SrTiO3 (Nb:STO) using low-temperature (LT) STM/S at 4.6 K in ultra-high vacuum (UHV). Upon in situ transfer to the LT-STM, the as-deposited samples with less than 1 u.c. show surface morphology with Ni:STO islands and bare Nb:STO spaces in between the islands, called trenches. Surprisingly, the trenches of the 0.5 u.c. sample already show specific patterns that can be corresponding to the nanocolumn patterns on a thick film, similar to vertically aligned nanostructures (VAN) morphologies observed in the literature.8 Film thickness of about 4.1 Å (lattice constant of STO~3.9 Å) is observed on both the 0.25 and 0.5 u.c. samples. Above the monolayer Ni:STO, homogeneously dispersed nanoparticles with diameters ranging between 2 and 3 nm exhibit low-density distribution. Local electronic properties of the nanostructures are investigated by performing STS and dI/dV, which yield spectra proportional to the local density of states. A bandgap of about 3.4 eV is seen on the Ni:STO film (tip-induced band bending takes part in the STS measurement), while the nanoparticles show a similar bandgap with extra sharp states 1.1 eV above the Fermi level, indicating increased p-doping concentration in the oxide nanoparticle. The STS on the Nb:STO substrate reveals a broader bandgap, exceeding 4 eV, attributed to the formation of Sr vacancies creating a space charge at the surface region9.
The study presents the very early stage formation process and the dopant distribution of the embedded nano-phase Ni:STO, allowing for improved control of such nanostructures, likely also relevant to understand the nucleation of VANs.
Abbey-K1
To mitigate the climate change and reach a carbon neutral society before it is too late, a mix of sustainable energy technologies are needed.
Batteries will continue to play a vital role in decarbonising transportation as well as in storing the intermittent renewable energy. Li ion batteries have revolutionised the electrification of transportation and contributed significantly to grid storage. However, there are increasing concerns with the availability of the minerals currently used in Li-ion batteries today, especially looking at the predicted growth of batteries demand. Diversification of battery technologies with more sustainable options in mind, not only for the raw minerals used in future batteries but also for more sustainable manufacturing practices for cells and packs are needed.
In my talk I will touch on some of these sustainable practices needed to be implemented today while showing the 12 principles of “green batteries” inspired from “green chemistry” my research group introduced. I will than focus on Na-ion batteries, the next battery technology in line for commercialisation in 2024, with emphasis on our research on hard carbon anodes on understanding the fundamentals on Na ion storage using a mix of characterisation techniques coupled with electrochemistry. I will also discuss the importance and complexity of solid electrolyte interfaces and some perspectives on commercialisation from our group.
I will also resent some new insights onto a very old research topic: intercalation of alkali metals into graphite. We have performed in depth study on K intercalation in graphite using a combination of Raman (including looking at the low frequency band), XRD, dilatometry, optical microscopy as well as DFT calculation which when coupled with electrochemistry and post-mortem TOF-SIMS reveal the formation of a solid electrolyte interface responsible for capacity loss in the first cycles.
I will present also on Al-ionic liquid-graphite dual ion batteriey configurations while focusing on the Al anode corrosion and degradation using XPS, XAFS and post-mortem microscopy after various cycles as well as understanding the intercalation of AlCl4- in graphite, soft and hard carbon and the difference in the storage mechanism among such classes of carbons.
Abbey-O1
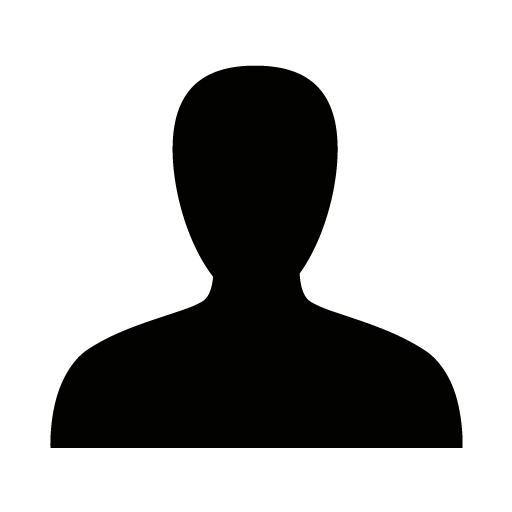
Today, state of the art lithium batteries are based on liquid electrolytes, due to their high ionic conductivity at room temperature. However, these batteries still exhibit shortcomings related to safety, durability and complexity of manufacturing or maintenance. For these reasons, all-solid-state lithium-metal batteries, employing solid electrolytes, have gained attention in recent decades due to their enhanced safety, chemical stability, and thermal stability [1,2]. Among solid electrolytes, ceramic ones offer superior chemical and thermal stability, resistance against dendrite growth, superior mechanical properties and wide electrochemical window. Despite their potential, their full deployment is still hindered by their performances and interfacial detrimental interactions with the electrodes [3,4].
Apart of the efforts of the scientific community at materials level, attention needs to be placed also at processing level. In this regard our group is exploring disruptive manufacturing processes for ceramic electrolytes and cathodes: 3D printing and ultra-fast high temperature sintering (UHS), in order to approach next generation of full ceramic devices.
In the present study NASICON-type Li1.5Al0.5Ge1.5(PO4)3 (LAGP) electrolytes were printed by stereolithography (SLA) in complex geometries difficult or sometimes impossible to produce with conventional ceramic forming techniques. Despite its benefits, only a limited number of studies have utilized 3D printing in the production of full ceramic electrolytes [5,6]. SLA is a promising method that allows the fabrication of intricate shapes with high resolution [7]. Here we present the development of SLA processes to produce LAGP full-ceramic electrolytes exploring two different geometrical approaches: the conventional flat electrolyte and a corrugated one which offer an increased interfacial area with electrodes thus leading to a reduced area specific resistance with the same projected area respect to its flat counterpart. Self standing LAGP structures were printed, debinded and sintered. Crack-free LAGP electrolytes with flat and corrugated geometries were succesfully produced with high densification (>80%), and uniform shrinkage in all directions. Those electrolytes demonstrated to have conductivities in good agreement with LAGP manufactured with conventional techniques (10−5-10−4 S/cm) and no detrimental reactions or degradation due to the debinding step were detected. Furthermore simmetrical cells Li/LAGP/Li flat and corrugated were tested in plating/stripping galvanostatic tests up to 200h.
The printed electrolytes were also coupled with Co-free LiFePO4 (LFP) cathodes printed by robocasting and attached by conventional thermal treatments (600ºC, 4h, N2 atmosphere). Even if 3D printing of LFP cathodes by robocasting has been reported in literature before it was limited to Li-ion batteries (employing liquid electrolytes) [8]. Our approach will potentially open doors to a full-printed, full-phosphate ASSB.
Simultaneously, we are developing UHS for the same electrolyte and cathode materials. UHS was introduced in 2020 as a sintering technique able to densify ceramics in seconds, with the promise of significantly decreasing both the production times and energy usage respect to conventional time-consuming and energy hungry thermal processes [9]. This method also aims to mitigate degradation phenomena and minimize lithium losses [9-11]. Results on UHS of the raw materials and printed pieces will be presented. LAGP was sintered by UHS in less than 2 minutes reaching very high densification (88%) and ionic conductivities comparable with the ones obtained by conventional sintering (10−4 S/cm). Furthermore, LFP was successfully attached on LAGP electrolytes by this technique avoiding detrimental interfacial interactions. Finally, UHS was successfully applied to 3D printed LAGP electrolytes lowering the times needed for debinding and sintering from days to minutes.
In conclusion, we believe that the combination of 3D printing (performances enhanced by design) and UHS (limitation of Li losses and degradation phenomena) will pave the way to the next generation of Co-free full-phosphate batteries with complex geometries.
Abbey-O2
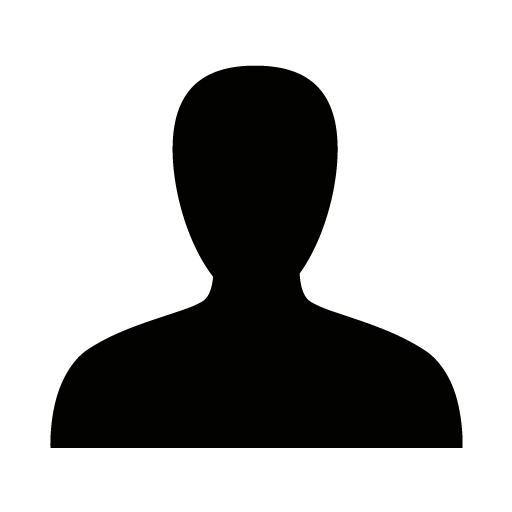
The replacement of conventional lithium-ion batteries with solid-state batteries is currently being investigated by a large number of players in both the academic and industrial sectors [1]. Sulfide-based electrolytes are among the materials considered most promising, especially for applications in the transport field [2]. For this type of solid electrolyte, manually assembled cells such as Swagelok cells, EL-CELLs and self-built pressure vessels are typically used to evaluate the performance of the anode, cathode and solid electrolyte materials [3]. However, coin cells are often out of consideration. Although coin cells cannot accurately predict how a material will perform in a battery cell format in an end-use application, they are easy to assemble and can provide reproducible data in comparison to the other cell types, making them an interesting option for testing materials under conditions that are more relevant to the intended application. This round-robin test for the preparation of solid-state coin cell batteries with a solid sulfide electrolyte, a lithium nickel manganese cobalt oxide cathode and a lithium metal anode is intended for academic researchers and provides guidelines for research in this field. The cell preparation method presented in this work has been evaluated for its reproducibility and can be modified depending on the parameters used to optimize the solid electrolyte, the cathode material, the bilayer consisting of cathode and solid electrolyte, the lithium metal anode and the cell in general. Defined electrochemical tests of a sulfide solid-state battery in coin cell configuration are measured in a round-robin test between four laboratories (see Figure 1).
Abbey-O3
see https://www.imw.uni-stuttgart.de/team/Clemens/
All solid-state fluoride ion batteries can be based on intercalation (e.g. La2NiO4) as well as conversion-based electrode materials (e.g., BiF3, CuF2, ZnF2, etc.) with solid electrolytes being typically tysonite-type of fluorite-type alkaline eart / rare earth fluorides (e.g. La0.9Ba0.1F2.9 or La0.6Ba0.4F2.6). If they want to compete with existing battery technologies, significant improvements in terms of cyclic stability are necessary to fully access the high specific capacities this battery concept can provide in theory.
In this contribution [1], we report the development of a high-pressure, high-temperature battery operation stand for battery cycling under inert conditions inside a glovebox. This stand was then used to investigate the effect of stack pressure on the cell performance of conversion-based as well as intercalation-based electrode materials for fluoride ion batteries. It was found that cyclic stability as well as energy efficiency is strongly increased compared to non-pressure conditions, which is assigned to sustained inter-particle contact. Thus, the cell-design must be considered carefully to be able to distinguish intrinsic material properties from percolation- and interphase-related impacts on the cell behavior. Further, the effect of pressure on the ionic conductivity of common solid fluoride ion conductors is investigated.
Gielgud-K1
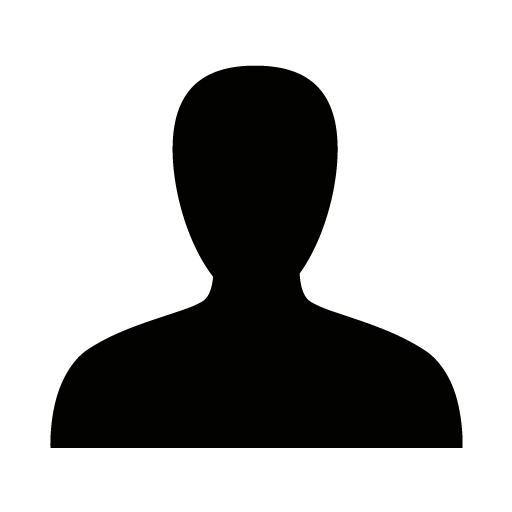
Ni-YSZ is the state of the art fuel electrode in solid oxide cells, but microstructural changes often occur near the electrode/electrolyte interface during operation that can cause performance degradation. This talk will review current experimental results and present phase-field simulations of microstructural evolution, with the initial structures obtained from measured three-dimensional Ni-YSZ microstructures. Ni migration and particle disconnection, as reported by some groups, is investigated by imposing a spatial gradient in Ni/YSZ interfacial tension. An analysis of the electro-wetting effect at Ni/YSZ interfaces is presented to explain the interfacial tension gradient. Both surface diffusion and capillary driven evaporation/condensation are explored as possible transport mechanisms; the latter is found to be too slow to explain observed Ni migration at normal operating temperatures. Effects of different Ni-YSZ starting microstructures, e.g., different Ni/YSZ ratios, are also explored. Results from analytical calculations of Ni migration velocity, assuming that the interfacial tension gradient arises from electro-wetting and that transport is via surface diffusion, are also presented.
Gielgud-O1
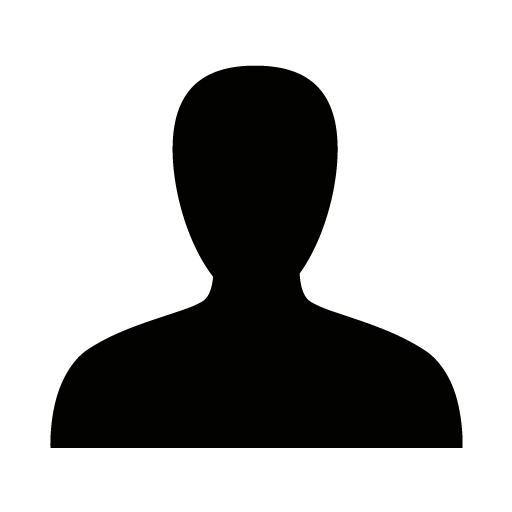
Seeking effective approaches for the efficient utilization of CO2 emission is desperately interested in the development of a sustainable community. Converting CO2 into highly valuable synthetic chemicals would be a promising way for energy storage. Using solid oxide electrolysis cells (SOECs) driven by renewable energy is expected to be one of the most efficient ways to convert electricity and industrial waste heat into chemical energy. Considering efficient energy conversion, recent development has focused on the simultaneous electrolysis of both CO2 and H2O in SOECs to directly produce industrially useful hydrocarbon feedstocks (e.g. H2/CO “syngas” mixtures) and fuels by means of well-established Fischer–Tropsch (F–T)-based processes in the chemical industry so that a “Power-to-Syngas” concept can be fulfilled. For practical application, developing a highly active fuel electrode with sufficient electrolysis current density and low degradation rate is an essential task for CO2/H2O co-electrolysis. In addition, to achieve the direct synthesis of useful fuels, controlling CO/H2 syngas product ratios from the feed gas of CO2/H2O is essential. However, the key challenge is particularly the significant effect of water gas shift reaction (WGSR) at relatively low temperatures (< 800oC).
In previous studies, we have discovered several potential fuel-electrode candidates, such as La(Sr)Fe(Mn)O3[1] perovskite and CuFe2O4[2] spinel for high-temperature CO2/H2O co-electrolysis. To facilitate their accessibility in intermediate temperatures; that is, particularly to suppress the influence of WGSR, their electrolysis performance with sufficient electrolysis current and appropriate syngas control is required to be further improved. Through an intuitive technique, infiltration of binary metal oxide,[3] fabricating active nanocatalysts over electrode matrix has shown intensively growing interest in designing highly efficient fuel electrodes for the application of CO2 and H2O electrolysis.
In this study, we investigated the influence of electrolysis performance by introducing various selected functional binary oxide catalysts such as lanthanide and transition metal oxides into the scaffold of the potential La(Sr)Mn(Fe)O3 and CuFe2O4 fuel electrodes by the infiltration technique for intermediate-temperature CO2/H2O co-electrolysis. We found that by the single- and multiple-infiltration of the selective oxides on these potential electrodes, significantly enhanced electrolysis current density and syngas product control can be achieved. For example, one of the particularly promising results shows a superior electrolysis current density (~2 A/cm2 at 800oC) can be achieved by Ce-infiltration, accompanying with markedly decreased internal resistances of the cells and exceptional Faradaic efficiency (nearly 100 %). The cell performance can also be tuned by the adjustments of infiltration concentrations and selecting multiple infiltrations of different binary metal oxides. In addition, syngas control can be significantly improved through varying oxide infiltrations and feed flow rates to increase CO2 conversion, resulting in a considerable suppression of water gas-shift reaction, and facilitating the operation in the intermediate-temperature range.
Gielgud-O2
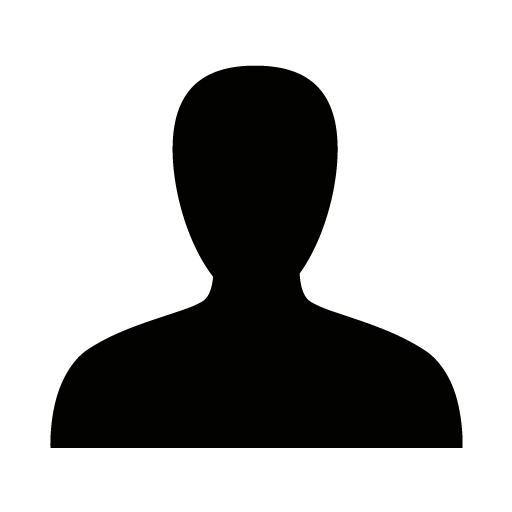
Composite materials based on molten salts and solid oxide phases have been suggested in the 90's as promising electrolytes for intermediate temperature fuel cells. The conductivity as a function of temperature presents a peculiar variation, with a huge gain of 2-3 orders of magnitude around the melting temperature of the salts. Conductivity close to 0.1 S.cm-1 can be reached at 500°C as in the case of Sm-doped ceria (SDC) associated to (Li,Na,K)2CO3 ternary eutectic composition. Our work aimed to go deeper in the determination of species and transport mechanisms in this electrolyte. We performed systematic studies as a function of temperature and gas compositions (oxidizing and reducing atmospheres, wet or dry) by impedance spectroscopy. DSC/TGA additional analyses, as well as post mortem X-Ray Diffraction were carried out. All results yielded to the important role of interfaces between molten carbonates and solid oxide phase, thus a partial transformation of carbonates into hydroxides in H2-rich atmospheres, accelerated in presence of water and avoided with CO2 addition [1]. This allowed us to define the atmospheric conditions for fuel cells / electrolysis operations, as well as the design of the electrodes. The reversibility has been highlighted with "symmetrical cells", as well as power density of 0.4 W.cm-2 at 0.7V in these conditions.
Gielgud-O3
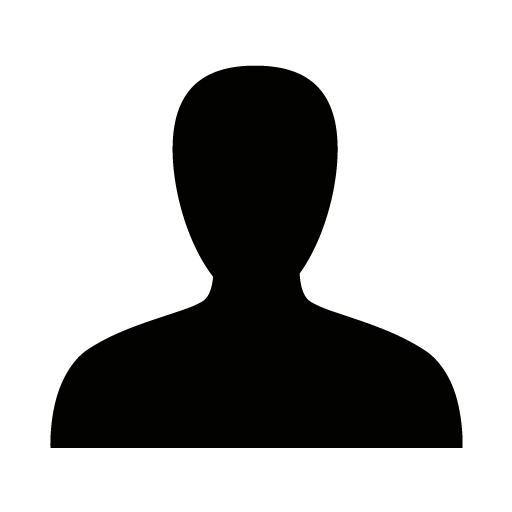
In this research, we address the critical challenge of fabricating a thin and dense Gd-doped ceria (GDC) buffer layer to impede interfacial elemental diffusion between the yttrium-doped zirconia electrolyte and the perovskite functional cathode within metal supported solid oxide fuel cell (MS-SOFC). To overcome the limitations of conventional sintering processes (>1400 ºC), we successfully synthesized GDC particles with a precise size of 3 nm using a novel precipitation method. The subsequent application of a spin-coating process enabled the formation of a thin (<1 μm) buffer layer at temperatures below 1000 ºC. Microstructure analysis at varying temperatures confirmed the efficacy of the synthesized buffer layers. These advancements were applied to a cutting-edge MS-SOFC, manufactured through co-firing in a reducing atmosphere. Electrochemical investigations, employing 2-probe AC impedance and DC measurements, highlighted the superior performance achieved through the optimization of microstructure in each layer and the incorporation of a thin ceria-blocking layer. This study presents a groundbreaking approach to enhance the efficiency and feasibility of MS-SOFC, emphasizing the significance of dense GDC buffer layer at low temperature and its application in next-generation metal-supported fuel cell technologies.
Gielgud-O4
The insufficient long-term stability of Solid Oxide Cells (SOCs) is one of the major drawbacks, limiting commercial usage of these electrochemical devices. The main reason for possible damage or degradation comes from the thermal expansion coefficient (TEC) mismatch between the electrode and electrolyte, which causes unwanted strain, cracks, and layer delamination. The problem may be mitigated by using negative thermal expansion coefficient materials (NTEs) that have been known for decades for their ability to decrease their spatial dimensions with increasing temperature. This interesting property along with the high electronic conductivity was recently reported for perovskite-based compounds like NdMnO3 and Sm1-xAxMnO3-δ (A = Zn, Cu; x ≤ 0.15) [1,2,3]. The addition of such materials to form composite-type oxygen electrodes for SOCs significantly lowers the TEC, adjusting it to the chosen electrolyte material, and diminishing possible degradation mechanisms. Therefore, the appropriately designed and produced composite electrode should possess suitably low polarization resistance, as well as is expected to deliver enhanced long-term stability, which in effect would greatly increase not only the performance but also the practical applicability of the SOC-based devices.
Double perovskite oxides from REBa0.5Sr0.5CoCuO5+δ (RE: Pr, Nd, Sm, Gd) group are known for their high mixed ionic-electronic conductivity, as well as suitable electrocatalytic activity, and are reported as attractive oxygen electrode material for SOCs. In this work, those materials were prepared by the typical sol-gel method or the electrospinning method. Among initially studied compositions, SmBa0.5Sr0.5CoCuO5+δ (SBSCCO) was found to be the best candidate, exhibiting demanded physicochemical and electrocatalytic properties. Also, this single-phase material is chemically stable and compatible with LSGM electrolyte. The total conductivity of SBSCCO is around 100 S·cm-1 between 300°C and 900°C, while if used as the oxygen electrode, the measured polarization resistance (Rp) in SBSCCO|LSGM|SBSCCO symmetrical cell was only 0.17 Ω·cm2 at 800°C. The long-term stability tests showed that the relative increase of Rp is around 20% after 5 days at 700°C.
To further enhance the characteristics of the SBSCCO electrode, selected NTEs: Sm1-xZnxMnO3-δ (SZMx) and Sm1-xCuxMnO3-δ (SCMx) were synthesized through the solid state reaction, with final annealing performed at 1300°C for 10 h in air. The conducted dilatometry measurements confirmed the negative TEC for those materials, ranging from -0.1·10-6 1/K to -11·10-6 1/K between in the 200-900°C range. Also, the total conductivity of SZM15 was found to be around 20 S·cm-1 at 900°C. Then, the previously obtained SBSCCO and chosen NTEs were used to prepare several composite oxygen electrodes, by adding different amounts of the respective powders. The preliminary results show that the Rp of the composite electrode with 10 wt.% addition of SZM15, as measured in the SBSCCO:SZM15|LSGM|SBSCCO:SZM15 symmetrical cell in the 700-900°C range, was significantly lower when compared with the unmodified SBSCCO electrode. For instance, Rp at 800°C was 0.12 Ω·cm2 giving a 30% relative decrease. Additionally, the I-V characteristics were measured for commercial anode-supported fuel cells comprising Ni-YSZ|YSZ support, GDC10 buffer layer, and the SBSCCO:SZM15 composite electrode. The maximum peak power density recorded at 800°C was 850 mW·cm-2, which is around 60% higher when compared to the same cell prepared with the SBSCCO electrode without NTE addition.
All the obtained results clearly show that the state-of-the-art innovative application of NTEs in SOCs indeed allows for decreasing the TEC of the composite and adjusting it to the solid electrolyte, but also enables a significant decrease of the polarization resistance. Further experiments are ongoing, regarding the long-term performance of the composite electrodes, SEM and TEM measurements involving the electrolyte/electrode interface.
Fleming-T1
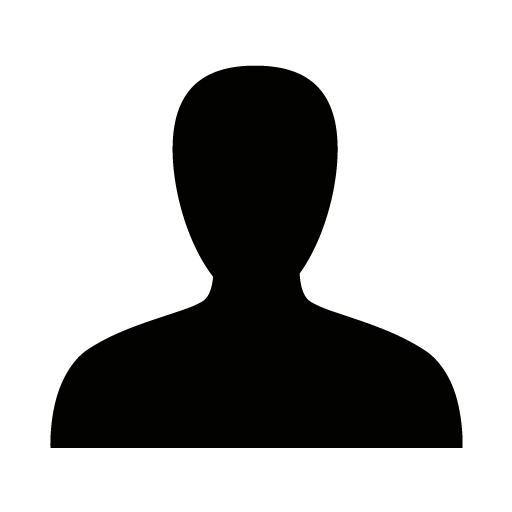
Recent years have seen monumental and exciting developments in the field of all-solid-state batteries (ASSBs). I will talk about several exciting scientific breakthroughs that have led to a renaissance of solid state chemistry. Despite its promises, solid state batteries still face a multitude of technical hurdles before commercialization can be achieved. Amongst these challenges, none are more daunting than the ability for scale-up prototyping, specifically, enabling technology transition from the laboratory to the pilot scale. A vast majority of ASSB reports to date are still limited to form factors impractical for actual device operation. In this plenary talk, I will provide a perspective on a wide range of scalability challenges and considerations for ASSBs, including solid electrolyte design and synthesis, dry electrode processing, cell assembly for optimum interfacial control, and stack pressure considerations at the cell level. More importantly, I will discuss ways to bridge the development gap between fundamental research and applications, through partnerships and collaborations between the industry, univeristies and national laboratories.
Fleming-K1
Arumugam Manthiram obtained his B.Sc. degree in chemistry in 1974 and M. Sc. degree in chemistry in 1976 from Madurai University and his Ph.D. degree in chemistry in 1981 from Indian Institute of Technology Madras. After working as a lecturer in chemistry at the Madurai Kamaraj University for 4 years and as a postdoctoral fellow both at the University of Oxford and at UT-Austin, he became a faculty member in the Department of Mechanical Engineering at UT-Austin in 1991. He is currently the George T. and Gladys H. Abell Endowed Chair of Engineering at the University of Texas at Austin (UT-Austin). He was the Director of the Texas Materials Institute and the Materials Science and Engineering Program at UT-Austin for 11 years during 2011 – 2022.
Dr. Manthiram's current research focuses on sustainable materials for batteries and fuel cells. Specifically, his group is engaged in developing new, sustainable, low-cost, efficient materials for batteries and fuel cells, novel chemical synthesis and processing approaches, and a fundamental understanding of their structure-property-performance relationships. He founded two startup companies: ActaCell Energy Systems in 2007 and TexPower EV Technologies in 2019. He has provided training and mentoring to ~ 300 students and postdoctoral fellows, including the graduation of 72 Ph.D. and 28 M.S. students. 60 of his students and postdoctoral fellows are faculty members around the world. He has authored 950 journal articles, 20 issued patents, and 10 pending patents. He has given more than 500 presentations, including 400 invited talks, and has edited 10 books. He has > 110,000 citations with an h-index of 162.
Dr. Manthiram is an elected Fellow of the National Academy of Inventors, Materials Research Society, Electrochemical Society, American Ceramic Society, Royal Society of Chemistry, American Association for the Advancement of Science, and World Academy of Materials and Manufacturing Engineering. He is an elected member of the World Academy of Ceramics. He received the university-wide (one per year) Outstanding Graduate Teaching Award at UT Austin in 2012, Battery Division Research Award from the Electrochemical Society in 2014, Distinguished Alumnus Award of the Indian Institute of Technology Madras in 2015, Billy and Claude R. Hocott Distinguished Centennial Engineering Research Award in 2016, Henry B. Linford Award for Distinguished Teaching from the Electrochemical Society in 2020, International Battery Association Research Award in 2020, Battery Division Technology Award from the Electrochemical Society in 2021, and Inaugural John B. Goodenough Award from the Electrochemical Society in 2023. He delivered the 2019 Chemistry Nobel Prize Lecture in Stockholm on behalf of Professor John Goodenough.
The anxiety for long driving range and for reducing the cost of lithium-ion cells is pushing to increase the nickel content in layered oxide cathodes. This is because Ni is less expensive and more abundant than Co. As the Ni3+/4+: 3d band barely touches the top of the O2-: 2p band, Ni3+ can be oxidized all the way close to Ni4+ without much oxygen loss from the layered lattice, resulting in capacities of as high as 240 mAh/g. In contrast, the overlap of Co3+/4+: 3d band with the top of the O2-: 2p band leads to an oxidation of O2- ions to oxygen beyond about 50% charge, resulting in a limited capacity of < 150 mAh/g. However, increasing the Ni contents above about 70% is met with a few hurdles: (i) The tendency of Ni3+ to get reduced to Ni2+ at the synthesis temperatures of about 700 oC necessitates a high stream of oxygen gas flow, introducing scale-up challenges for obtaining high quality materials with consistency. (ii) The instability of Ni4+ in contact with the liquid electrolyte in the charged state introduces aggressive surface reactivity, which results in rapid capacity fade and thermal runway. The surface reactivity is accompanied by loss of oxygen from the surface, reduction of Ni4+ to Ni2+ on the surface and formation of resistive rock-salt phases, formation of cracks, loss of active lithium in cathode-electrolyte interphase formation, Ni dissolution and migration to the anode due to the electronically driven lattice instability associated with the Jahn-Teller active Ni3+, and consequent capacity fade during extended cycling [1]. The reactivity also leads to thermal instability, oxygen loss, gas evolution, and safety concerns [2]. These challenges become exponentially aggravated with Ni contents > 80% and even much more for > 90%.
To overcome the challenges, generally doping of high-Ni cathodes with various dopants is pursued. However, no clear understanding is available in the literature on which dopant does what. Doping is generally carried out randomly in the literature by a trial and error process. This presentation will provide a systematic investigation of doping LiNiO2 with 5% or 10% of various common dopants like Co, Mn, Al, and Mg. The effect of dopants on capacity, cycle life, thermal stability, and gas evolution will be presented. The surface reactivity depends both on the surface characteristics of the high-Ni cathodes and the electrolyte. This presentation will then focus on the development of cathodes with a robust surface as well as more stable electrolytes to overcome the challenges. The ability to achieve long cycle life, while reducing the amount of gas evolution, by tuning the cathode and electrolyte compositions will be presented. Also, the fundamental understanding developed with the use of advanced analytical techniques, such as in-situ XRD, SEM, XPS, and TOF-SIMS will be discussed.
Fleming-I1
Francois Weill is CNRS Research Director at the Institut de Chimie de la Matière Condensée (ICMCB-CNRS, France) at the Bordeaux University. He gratduated (PhD) in solid-state chemistry at the University of Strasbourg in 1989. That same year, he joined the ICMCB in Bordeaux. Since then, he has been interested in the crystallographic study of materials by imaging and electron diffraction. In the "Energy and Materials" team, he studies the ordering in materials for the positive electrodes of Li(Na)-ion batteries whether they are lamellar materials, spinel type materials or poly-anionic materials. He is the co-author of more than 150 research paper. He is responsible for the electron microscopy center of the Aquitaine materials characterization platform.
The spinel material LiNi0.5Mn1.5O4 is a good candidate as a positive electrode material for Li-ion batteries, thanks in particular to its high energy density and the absence of cobalt. But the performance of this material is highly dependent on the stoichiometry and order of the two transition metals. These parameters are closely linked to the conditions under which the material is synthesized.
Molten salt synthesis has enabled us to obtain a multi-faceted platelet material for which, by varying the temperature and atmosphere of the heat treatment, it is possible to control the order of the transition metals in the absence of impurities detectable by diffraction [1]. The electrochemical properties of this platelet material are fully comparable to those of a material with a classical octahedral morphology.
Raman and NMR spectroscopies were used to explore the local order in these platelet materials. 4D-STEM was used to observe transition metal order at the particle level with a spatial resolution of 10 nm. By comparing two globally ordered materials with a difference in order homogeneity at the particle level, we were able to show that heterogeneity in transition metal order was beneficial to lithium mobility, enabling a globally ordered material to achieve electrochemical performance comparable to a disordered material.
Fleming-O1
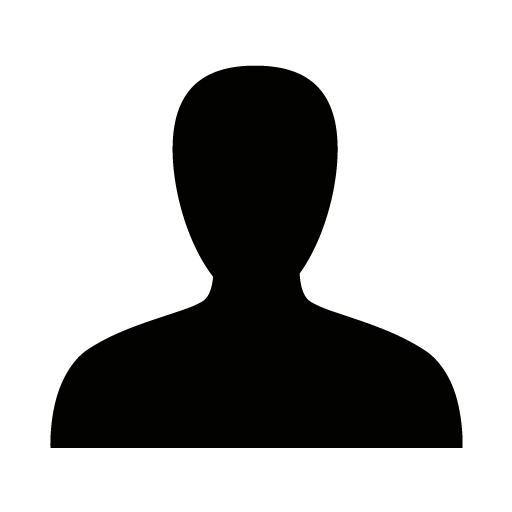
A large proportion of the batteries marketed worldwide are Li-ion batteries, which generally use a mixture of lithium salts and organic liquid as an electrolyte (LE). But this technology poses several issues, the main one being safety due to the high flammability of the organic solvent, and the energy density also reaches limits with LE since Li metal cannot be used as negative electrode. All solid-state batteries could provide a suitable alternative by replacing LE with a solid electrolyte that is less flammable and allows lithium metal to be used as a negative electrode to increase energy density [1]. The thiophosphate materials family is one of the most promising ones owing to their good ionic and suitable mechanical properties. However, the numerous solid-solid interfaces especially the electrode/electrolyte ones are complex to handle as it is one of the principal causes of electrochemical fading [2]. Especially on the positive electrode, the optimal contact between the two solids promotes the chemical decomposition due to the contact between an oxide (electronic conductor) and a thiophosphate (ionic conductor), leading to the creation of several insulating products that generate an increase in impedance. Composite electrode engineering is not an easy task due to the intrinsic engineering of the solid-state batteries where there must be no (or almost no) porosity after sintering to improve wetting between the active material and the electrolyte. Indeed, the contact is the sole option to guarantee an optimized electrochemical activities and transport properties [3]. Here, we proposed to investigate an Li6PS5Cl/NCM523 composite electrode prior cycling to understand the impact of the electrode engineering such as the ratio of the solid electrolyte/electroactive materials, the nature of the electronic/ionic conductor. The investigation will be carried out by means of electrochemical impedance spectroscopy performed at different temperature coupled with several advanced characterizations performed at ESRF synchrotron (XRD, XAS). The goal being to find the optimal ratio to ensure a proper ionic and electronic conductivities while giving satisfactory electrochemical performance.
Westminster-K1
The ability to design structures that can regulate and control the ionic functionalities of electroceramics for applications in a wide range of devices requires a fundamental understanding of transport pathways. Ionic transport typically takes place in the presence of a wide range of defects including dopant species, dislocations, precipitates, grain boundaries, and surfaces. The ability to elucidate the relationship between local structure and transport remains an ongoing challenge for both experimental and computational techniques. Modern transmission electron microscopy (TEM) allows the structure and composition of electroceramics to be characterized down to the atomic level. However, the dream of in situ electron microscopy is to characterize the transport and reactivity functionalities with high spatial resolution. Can transport/diffusion processes be directly observed at the atomic level? What does such a statement even mean?
Molecular timescales are typically in the picosecond range and atomic level information is available with advanced computational methods like ab initio molecular dynamics(Kang, Vincent et al. 2022). But large-scale complex systems with processes involving correlated atomic motions remain computationally inaccessible. Experimental ultrafast approaches to high resolution TEM can yield time resolutions of picoseconds, at least for cyclic processes, but the associated spatial resolution is limited to 2 nm (Zhang and Flannigan 2019). Bridging this spatiotemporal gap is an ongoing challenge in the field. An emerging new generation of electron detectors have greatly improved sensitivity and readout rates allowing atomic resolution information to be captured on the millisecond time scale. While this remains far from molecular timescales, it does make it possible to consider looking for repetitive atomic dynamic processes, such as diffusion pathways, that may be matched to millisecond temporal resolution(Lawrence, Levin et al. 2020, Lawrence, Levin et al. 2021). The rate of structural dynamics can be manipulated and matched to the system readout speed through temperature control. For example, a process with an activation energy of 0.6 eV will manifest about 1000 jumps per second at room temperature. This lies within the range of activation energies for oxygen migration pathways in many oxygen ion conductors.
Here we use aberration corrected in situ transmission electron microscopy equipped with cutting edge detector technology to explore ionic transport and diffusion in oxides with a focus on cerium dioxide and strontium titanate. Oxygen transport is easier to detect, at least in comparison to say, lithium transport, because of the larger anion size and the significant perturbation of the cation sublattice during transport. However, even at millisecond time resolutions, the signal per frame gets very small for atomic resolution analysis with moderate electron dose rate. To compensate for this, we have developed convolutional neural networks to denoise data sets (Marcos-Morales, Leibovich et al. 2023). With oxygen transport, local short-lived structural changes can be rather subtle to detect. Consequently, novel sophisticated image processing techniques must be employed to characterize the nature of the structural dynamics (Thomas, Crozier et al. 2023). This presentation will review what is currently possible in measuring atomic resolution structural dynamics in electroceramics and how the field is likely to evolve over the near future.
Westminster-I1
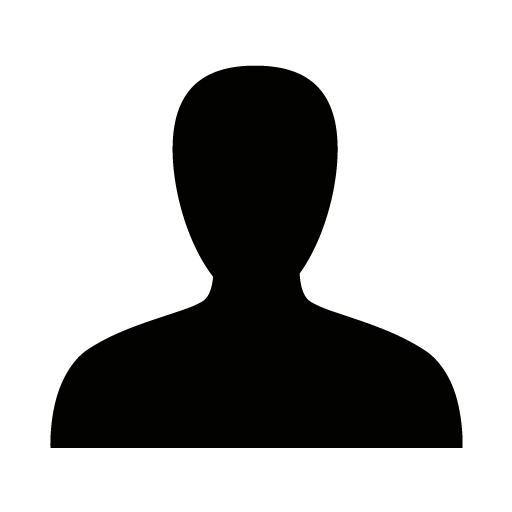
In a search for new, improved mixed ionic-electronic conductors (MIEC) exhibiting a desired set of structural, thermomechanical, transport, and electrocatalytic properties, various complex perovskites and related oxides have been proposed i.a. as excellent oxygen electrode materials for Solid Oxide Cells (SOCs). Apart from their relatively complex and often highly anisotropic structural features, usually manifested through formation of various superstructures, such oxides may also show compositional complexity, with the emerging group of the high entropy materials being developed as well. In this work several systems are described in more details, including the A-site layered RE(Ba,Sr)Co2-yMnyO5+δ (RE: selected rare-earth cations; 0 ≤ y ≤ 2), for which, depending on the Mn content, the physicochemical properties can be optimized for the SOC, or alternatively, for oxygen storage-related applications [1, 2]. In another group, RE(Ba,Sr)Co2-yCuyO5+δ, doping with copper is found to occur in a limited range, however, materials at the limit of formation of solid solutions may exhibit enhanced electrocatalytic properties [3]. Together with the appropriate other characteristics, as well as significantly decreased cobalt content, they appear as very good candidates for SOC oxygen electrodes. Importantly, depending on the chemical composition and preparation method (e.g. by electrospinning), some of those oxides can be obtained in a single perovskite structure, showing altered properties in comparison to their respective double perovskite counterparts. Also, pure Cu-based perovskite-related oxides with a general formula of La1-x(Ba,Sr)xCuO3-δ are found as very promising candidates for SOC oxygen electrodes, however, possibility of obtaining single phase compounds is relatively limited in the considered system, and often, the oxygen sublattice shows strong ordering, which hinders the ionic conductivity [4]. In this aspect, finding systems with the (partially) disordered oxygen vacancies is reported as beneficial, especially regarding electrocatalytic activity at moderate temperatures [5]. In another approach, multicomponent at either the A- and/or B-site perovskites have recently become of interest, however, the actual role of the high entropy in terms of influencing the properties (in terms of variation from the expected solid solution-based rules) is not yet understood [6]. Finally, the (small RE cation-based) REMnO3-type hexagonal oxides, with compositions close to the perovskite-hexagonal phase transition border, can be optimized for the temperature swing absorption processes, which enable oxygen separation from air at relatively low temperatures of 200-300 °C [7]. The oxides show unique set of the physicochemical properties, including presence of interstitial oxygen defects. This work is summarized with several guidelines, which should be helpful for designing and obtaining such complex oxides exhibiting the desired useful properties.
Westminster-O1
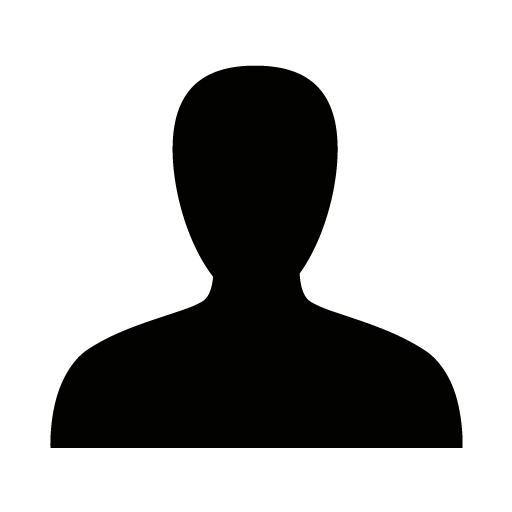
The technology of solid oxide fuel cells (SOFCs) is widely considered to be one of the essential components of the next-generation power grid, offering unmatched efficiency, low pollution level, and the possibility of both producing (fuel cell mode) and storing energy (electrolysis mode). Its main deficiencies are high operating temperatures and still limited longevity, both factors being affected primarily by insufficient performance and degradation of the cathode materials. Unfortunately, in most state-of-the-art solutions, the excellent performance of materials walks hand in hand with their susceptibility to deterioration under cell’s operating conditions. A common reason for this behavior is the presence of alkali ions, mainly Sr and Ba, which on the one hand are considered indispensable for achieving sufficient catalytic activity, but on the other tend to form secondary phases, such as carbonates, which over time limit the amount of active cathode sites. One of the possible solutions proposed to counter these effects is the application of the high-entropy approach, which enables obtaining properties beyond the rule-of-mixture, and therefore overcoming some of the limitations of more conventional analogs. However, strict adherence to the high-entropy guidelines might become a limitation by itself, as the more elements we combine, the more difficult it becomes to preserve the single-phase structure and avoid the unwanted interactions between them.
In this study, we present the possibility of obtaining highly functional, alkali-free perovskites of superior performance, obtained through the progressing simplification of the starting La-Co-Cu-Fe-Mn-Ni and La-Cu-Fe-Mn-Ni-Ti high entropy systems. The properties of all materials are systematically studied, including their structure, oxygen nonstoichiometry, thermal expansion behavior, electrical properties, and stability against both typical electrolytes and under the operating conditions of the fuel cell. For the selected, most promising materials, the level of electrochemical performance is assessed using both symmetrical cells (cathodic polarization value Rp) and full cells (power output). The results show that most of the beneficial properties characterizing the base, high entropy systems, can be preserved in simpler, 3- and 4-component compositions while at the same bringing several new features, thanks to eliminating some of the suboptimal elements from the mixture. The electrochemical performance of the best materials, such as La(Co,Cu,Fe)O3 and La(Cu,Fe,Ni)O3, despite the lack of alkali ions is superior to the state-of-the-art LSCF (La0.6Sr0.4Co0.2Fe0.8O3-δ) by a considerable margin, reaching the cathodic polarization value of 0.15 Ω·cm2 in the vicinity of 675 °C, making these materials potential candidates even for the low temperature SOFCs (LT-SOFCs). This is especially impressive for the La(Cu,Fe,Ni)O3 composition, which not only does not contain alkali ions, but cobalt as well. Overall, it can be stated that the proposed approach can lead to materials characterized by a superior combination of functionality and electrochemical performance with respect to the currently utilized alternatives, while simultaneously addressing some of the most pressing issues of the SOFC cathode materials.
St.James-K1
Chemo-mechanical durability challenges in electrochemical applications, arising from chemically-induced strains, drive the search for near-zero-strain materials. We focus our studies on ABO3 perovskite oxides as prototype structures, evaluating the impact of B-O bond features on redox and hydration coefficients of chemical expansion (CCEs). CCEs normalize the chemical strain to the stoichiometry change, and our goal is to enable efficient design of near-zero-CCE materials through uncovering crystal chemical descriptors. We combine in-situ neutron diffraction, X-ray diffraction, dilatometry, and thermogravimetric analysis, with ex-situ X-ray absorption spectroscopy and DFT simulations to quantify CCEs and correlate structure and behavior across multiple length scales. Contrary to expectations, when considering a wide enough range of zirconate and cerate proton-conducting compositions, hydration CCEs do not scale monotonically with lattice parameter nor with chemical strain anisotropy. Instead, we have found that there appears to be a minimum in hydration CCE for perovskites with intermediate octahedral tilt angles, which can accommodate hydration through the largest decreases in B-O-B angles. This mechanism does not significantly enlarge the lattice parameters. For redox CCEs, the charge distribution in the B-O bonds of mixed ionic/electronic conductors plays an important role. While we have observed minimal redox CCEs for systems where redox takes place fully on the anion rather than the cation, we also sought to quantify the link between octahedral tilt angles, hybridization, and CCEs. When comparing a limited set of ferrites where tolerance factor is varied by changing the A-site cation, lower CCEs correlate to both lower B-O-B angles and higher degrees of hybridization. Ongoing work expands these studies to a wider compositional range with other B-site cations to verify these relationships.
St.James-I1
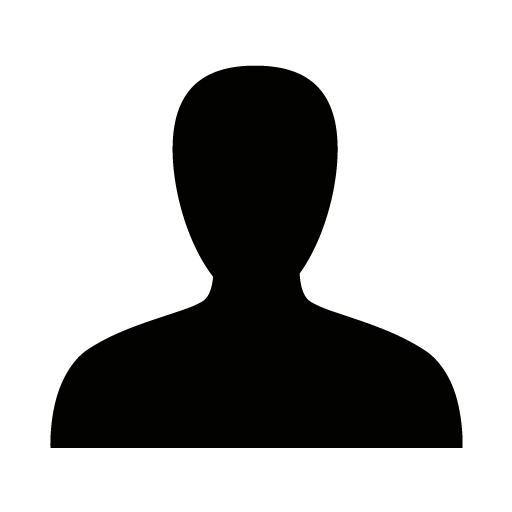
Triple-conducting oxides, which conduct oxygen ions and electrons or holes as well as protons, are a promising option for electrodes of protonic ceramic fuel and electrolyser cells (PCFCs, PCECs), as the reaction zone extends beyond the three-phase boundary of the air electrode, increasing the rate of oxygen reduction. However, for single-phase triple-conducting perovskites, a conflict between electronic conductivity and proton uptake has been observed [1].
The present study focuses on self-generated Ba(Ce,Fe,In)O3-δ composites of Ce-rich and Fe-rich perovskites that collectively satisfy the desired properties for proton uptake and transport properties of air electrodes in PCFCs and PCECs. In the system BaCe0.5Fe0.5O3-δ [2], In3+ is substituted on the B-site to induce oxygen vacancies and increase the hydration capacity. Crystal structures, lattice parameters and the relative amounts of the formed phases were determined by X-ray diffraction. The local chemical composition (cation distribution) within the individual phases, which is decisive for the transport properties of the composite, was determined by scanning transmission electron microscopy including energy-dispersive X-ray spectroscopy. Proton uptake was analysed by thermogravimetry.
Annealing experiments showed that the miscibility gap of the BaCe0.8-xFexIn0.2O3-δ system ranges from [Ce]/([Ce]+[Fe]) ratios of approximately 0.2 to 0.9 and narrows with increasing dopant concentration. The acceptor In3+ tends to accumulate in the Fe-rich phase, similar to Y3+ in the Ba(Ce,Fe,Y)O3-δ system [3]. The ratio of In(Ce-rich phase) / In(Fe-rich phase) ranges from 0.3 to 0.7.
The proton concentrations of the Ba(Ce,Fe,In)O3-δ composites are within the range of 1-4 mol% at 400°C. The proton uptake increases with higher amounts of In and lower amounts of Fe in the precursor in the composite samples as well as in Ce- or Fe-rich Ba(Ce,Fe,In)O3-δ single phases. Measurements on BaCe0.4Fe0.4Acc0.2O3‑δ (Acc = Y, Yb, Gd, Sm, Sc) composites show a general increase in proton uptake compared to the undoped system BaCe0.5Fe0.5O3-δ [4]. The variation of the acceptor ion has only a minor effect on the proton uptake. The driving forces for the dopant accumulation in the Fe-rich phase such as size mismatch and acid/base properties will be discussed.
St.James-O1
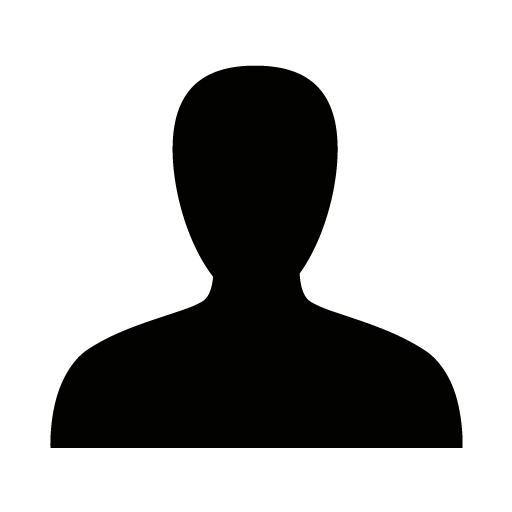
Electrochemical conversion from fuel to electricity and vice versa, with high efficiency, is a key technology for achieving carbon neutrality. Electrochemical devices that operate at intermediate temperatures of around 300°C are an undeveloped technological area and thus offer promising possibilities for new applications. Particularly, in terms of catalytic reactions, the intermediate temperature range is expected to provide better control over product selectivity and reaction activity compared to low-temperature or high-temperature devices. Currently, only a few electrochemical devices for CO2 conversion in this temperature range have been reported, utilizing CsH2PO4 proton conductors as electrolytes [1,2].
In this study, instead of CsH2PO4, which lacks long-term stability, a new type of electrochemical cell was fabricated using a phosphate glass proton conductor developed by alkali-proton substitution (APS) method [3,4] as the electrolyte in combination with a cermet-type porous substrate.
Previously, single cells for fuel cells were fabricated by hot pressing glass electrolyte blocks onto Pd plates [5]. However, for practical reasons, such as cell scale-up and cost considerations, it became necessary to develop a cell with an electrolyte fabricated on a conventional support. Therefore, we developed a process to form a glass electrolyte on a porous cermet, similar to those used in SOFCs and SOECs. The glass electrolyte, with a composition of 36HO1/2 - 4NbO5/2 - 2BaO - 4LaO3/2 - 4GeO2 - 1BO3/2 - 49PO5/2 [3], was prepared by APS method and then powdered for cell fabrication. This powder was coated on a porous Ni-Gd0.1Ce0.9O1.95 cermet support (φ18mm), and a dense glass electrolyte layer was formed by hot-pressing at 330oC. The electrolyte layer's thickness, around 40μm, was confirmed by cross-sectional SEM observations. The proton conductivity of the glass electrolyte remained consistent post hot-pressing, measuring 2x10-3 Scm-1 at 300°C under H2 atmosphere using a Pd sputtered film electrode (φ10mm). This demonstrates that the powdering and hot-pressing procedure did not affect the proton conductivity. We successfully fabricated a cermet-supported type phosphate glass electrolyte electrochemical cell. To confirm its potential in CO2 reduction reactions, experiments were carried out using cermet-supported type cell employing Ru fine particles as the electrocatalyst. 100% H2 gas was supplied to the anode and 100% CO2 gas to the cathode at 300oC and the exhaust gas was analyzed by gas-chromatography. When a voltage of 5V was applied, corresponding to the direction of proton transport from the anode to the cathode, hydrogen production was observed, and CO and CH4 gases were also detected. Faraday efficiencies for each gas production were 19%, 12% and 68% for H2, CO and CH4, respectively. The high Faraday efficiency for CH4 production indicates that the supplied H2 gas is being efficiently utilized in the reaction with CO2, and this result proves that our cell can be effectively used for CO2 reduction. The ability to electrochemically control the potential of reactants opens up the possibility of developing a new field of research in reaction chemistry using ionic conductors. Currently, we are carefully examining whether an electrochemical reaction acceleration effect is being observed.
Moore-K1
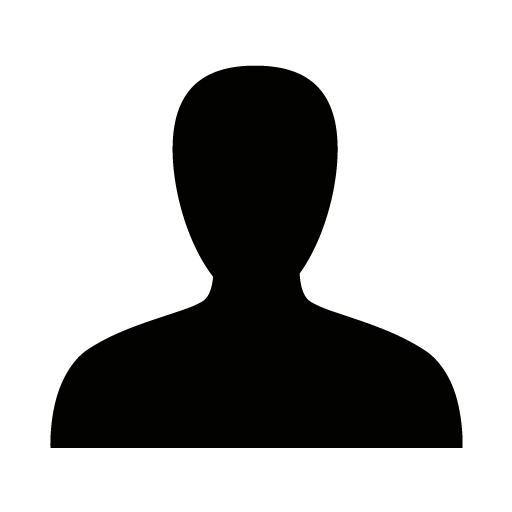
The evolution of materials under irradiation is driven by the enhanced transport of atoms during irradiation. To understand, predict, and ultimately design new materials destined for nuclear energy applications, it is imperative to understand the motion of these atoms. Currently, there are no probes to directly measure this transport during irradiation. Electrochemical impedance spectroscopy (EIS) has been extensively applied to these types of materials from the point of view of their conductivity and functionality. Los Alamos National Laboratory (LANL) recently established the capability of combining electrochemical measurements with ion beam irradiations to develop a first-of-its-kind capability to directly measure the transport of atoms during irradiation. Different oxides have propensity for being radiation tolerant either through rapid self-annealing of radiation induced defects or through the formation of meta-stable disordered structures or phase changes. The current work explores the changes in conductivity under light ion irradiation of oxide materials with varying levels of radiation tolerance.
Moore-O1
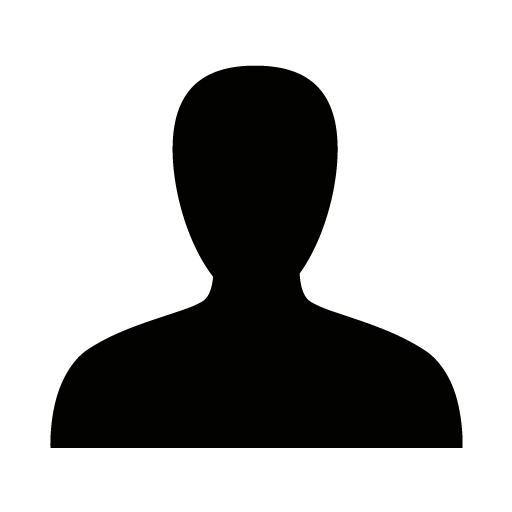
Solid Oxide Electrolyser Cells (SOEC) can achieve unrivalled efficiency in converting renewable electrical energy to hydrogen and therefore are an indispensable part of our transition to a sustainable energy economy, but the technology is not yet fully developed. One approach to improve the performance of SOEC is using gadolinia-doped ceria (GDC) as electrolyte, since its higher ionic conductivity compared to the current standard, yttria-stabilised zirconia (YSZ), allows significantly higher electrolysis currents at a given voltage. However, GDC suffers from electrochemical expansion upon reduction; with pO2 as low as 10–19 Pa at the fuel electrode-electrolyte interface, this expansion can lead to cell cracking, limiting its lifetime. In order to design a cell that can withstand such effects, it is necessary to precisely characterize the electrochemical expansion under cathodic bias. To achieve this, we have developed an in situ cell for investigating the electrochemical expansion of GDC at relevant temperatures, in reducing gas atmosphere and with applied cathodic bias simultaneously. In our setup, the SOEC is mounted between the air compartment, which houses a heater, and the fuel compartment, which features an X-ray window for diffraction and spectroscopy. The cell is electrically contacted from both sides to monitor the cell voltage and determine the cathode overpotential. In conjunction with the X-ray techniques, operational temperature of up to 800 °C and separate air and water/hydrogen atmospheres for the air and fuel electrodes, allow conducting operando experiments on real working SOEC.
Moore-O2
Modern MEMS-based transmission electron microscopy (TEM) holders enable precise specimen environment control, which is ideal for direct in-situ and in-operando observations, whether in real time or not. This talk presents two examples where MEMS-based holders are used primarily to control material processing and chemical reaction progress, enabling unique experiments assessing (1) solid-phase crystallization of a mixed ion-electron conducting (MIEC) amorphous complex oxide—a model amorphous low-temperature solid oxide fuel/electrolysis cell electrode, and (2) CaCO3 calcination—a model solid-vapor decomposition reaction and the critical step in calcium looping for CO2 capture and thermochemical energy storage.
Key aspects of MIEC amorphous complex oxide processing such as the evolution of structure/chemistry with temperature and the corresponding effect on electrical conductivity are detailed, and approaches to direct amorphous complex oxide processing towards desirable synthesis products based on these insights are discussed, applied to the amorphous La0.8Sr0.2MnO3-δ system [1]. To elucidate the CaCO3 calcination reaction, we applied correlated in-situ electron and synchrotron probes to directly observe CaCO3 calcination and report a new reaction mechanism involving heterogeneous nucleation of nanoporous CaO at CaCO3 grain boundaries followed by planar reaction front motion, in contrast to the widely accepted spherical shrinking core model [2].
[1] Jenna L. Wardini, George Harrington, Sangjun Kang, Dennis Kemp, Roger A. De Souza, Christian Kübel, William J. Bowman. (In revision).
[2] Jenny Martinez, Jenna L. Wardini, Xueli Zheng, Joann-Matthew Means, Huiming Guo, Pratik Dholabhai, Leora Dresselhaus-Marais, William J. Bowman. (2024) Advanced Materials Interfaces.
Abbey-K1
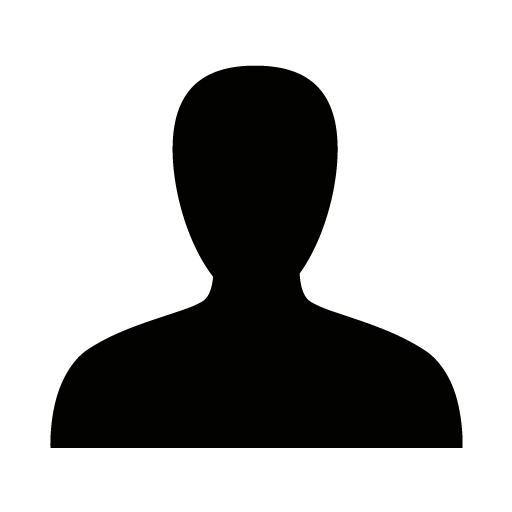
The targeted variation of oxide material properties by changing their composition is an integral part of everyday scientific work in the field of solid state ionics. This approach can be used, for example, to change the conductivity of an oxide for a specific charge carrier species, to influence the space charge that forms at an interface, or to manipulate the surface reaction kinetics of an electrode. The latter in particular has attracted a great deal of attention in recent years in several respects. On the one hand, the enormous variability of the reaction kinetics of mixed-conducting oxide electrodes has been linked to small changes in their surface chemistry, which has also opened up the possibility of compensation strategies for degradation phenomena. On the other hand, the concept of exsolution (which involves the intentional partial reductive decomposition of perovskite-type materials forming catalytically active surface particles) has emerged as an exceptionally compelling tool for preparing novel heterogeneous catalysts with highly attractive properties. What can be learnt from the investigation of such highly surface-sensitive systems is that materials with completely new electro-catalytic properties can be generated by applying only small changes that have their effect primarily on the surface.
In this contribution a number of various possibilities of manipulating the surface chemistry of mixed conducting electrodes will be explored:
(i) The perovskite-type electrode material (La,Sr)FeO3-δ (LSF) is modified by application of tiny amounts of platinum. First, as a dopant by introducing Pt during the synthesis procedure of the oxide. This yields the remarkable result that Pt in LSF has no extra catalytic activity, but nevertheless enhances the electrode’s oxygen exchange kinetics. This at first glance peculiar behavior, however, can be explained by an influence of the noble metal dopant on the available number of redox-active sites. Second, Pt is applied as nanosized, metallic surface particles. In this form, its effect is completely different with the p(O2) dependence of the oxygen reaction being even inversed compared to bare LSF.
(ii) The complex behavior of exsolution particles under electrochemical polarization of the corresponding parent oxide is highlighted. More specifically, the oxidation state and the associated catalytic activity of particles exsolved from ferrite-based thin-film electrodes are selectively switched by applying a bias voltage. Interestingly, in case of iron particles, the oxidation state of the particles is almost exclusively governed by the parent oxide, while nickel particles are significantly more influenced by the atmosphere. We explain the behavior with a 'kinetic competition' between the gas atmosphere and the applied electrochemical driving force with different oxygen transport kinetics in Fe- and Ni-based particles as a decisive factor. Additionally, we demonstrate voltage-controlled sequential exsolution of Ni and Fe nanoparticles, and extend our exploration to bimetallic exsolution systems with three distinct activity states.
Abbey-I1
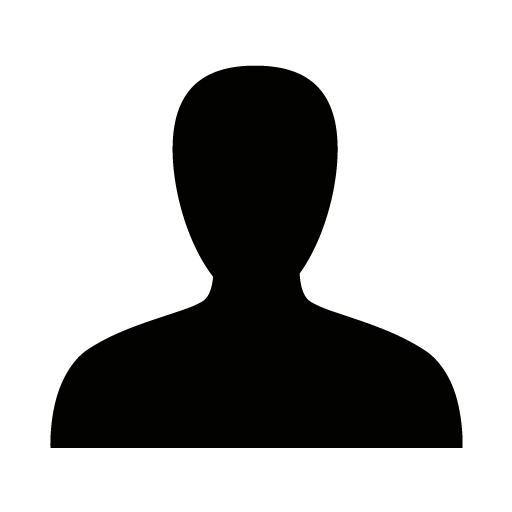
Dynamic structural and chemical changes of catalyst materials under operation conditions play a crucial role for the activity and stability of electrocatalysts. It is essential to understand the mechanistic processes that govern the material response, oftentimes particularly affecting the catalyst surface and therefore the electrochemical interface, to improve the lifetime of energy conversion devices such as water splitting catalysts or solid oxide cells. Exploring the underlying effects may further allow to develop design principles for tailoring the functionality of energy materials.
Metal exsolution reactions can be driven in fuel electrode materials under the reducing operation conditions of solid oxide cells. The process enables the synthesis of nanostructured catalysts based on the release of a fraction of reducible dopants from a perovskite host to its’ surface and the subsequent nucleation of finely dispersed oxide-supported metal nanoparticles. The performance of such catalysts strongly depends on the nanoparticle characteristics, such as the nanoparticle size and nanoparticle density, correlated to the properties of the active triple-phase-boundaries.
We employ epitaxial thin films with atomically defined surface morphologies to study the exsolution kinetics with respect to the mass transport of Ni dopants from the bulk to the perovskite surface. In addition, we investigate the subsequent growth and coalescence behavior of the exsolved nanoparticles during the reducing thermal annealing. We demonstrate that the electrostatic interactions of exsolution-active dopants with the surface potential that is correlated to the inherent surface space charge region of perovskite oxides determines the kinetics of metal exsolution in our material system [1]. Moreover, we reveal that defects that form under the reducing reaction conditions at the surface of the perovskite support can accelerate nanoparticle clustering and hence degradation of metal exsolution catalysts [2]. Based on our findings, we derive strategies for the control of the metal exsolution dynamics and for the stabilization of exsolved nanoparticles.
Abbey-O1
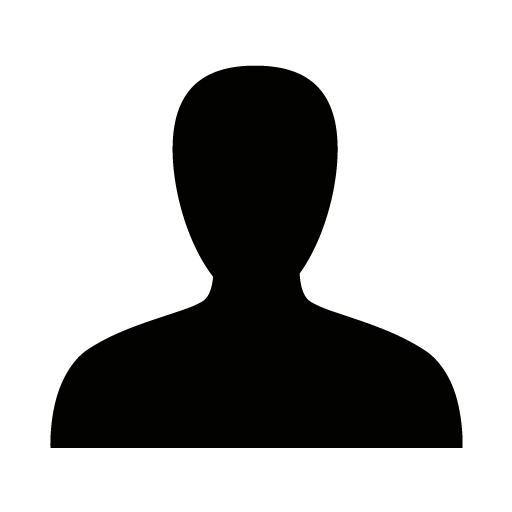
In situ exsolution has emerged as an outstanding route for producing oxide-supported metal nanoparticles. A variety of transition-metal cations can be incorporated into the host lattice – typically a perovskite-oxide – under oxidising conditions and exsolved as metallic nanoclusters after reduction. Consistent and comprehensive descriptions of the thermodynamics and kinetics of this phenomenon are lacking, however.
Herein, supported by hybrid Density-Functional-Theory calculations, we propose a single model that explains diverse experimental observations; why transition-metal cations (but not host cations) exsolve from perovskite lattices upon reduction; why different transition-metal cations exsolve under different conditions; why the metal nanoparticles are embedded at the surface; why the oxide’s surface orientation affect behaviour; why exsolution occurs surprisingly rapidly at relatively low temperatures; and why the re-incorporation of exsolved species involves far longer times and much higher temperatures. Our model’s foundation is that the transition-metal cations are completely reduced within the perovskite lattice as the Fermi level is shifted upwards within the bandgap.[1] The calculations also emphasise the importance of oxygen vacancies and A-site vacancies. Our model provides a fundamental basis for improving existing, and creating new, exsolution catalysts.
Gielgud-K1
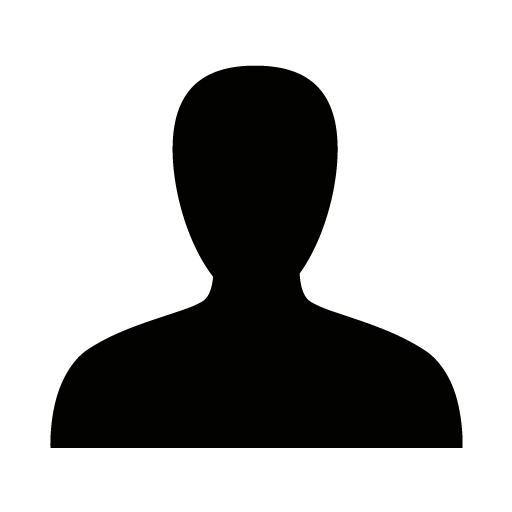
The need for new materials to tackle societal challenges in energy and sustainability is widely acknowledged. As demands for performance increase while resource constraints narrow available options, the vastness of composition, structure and process parameter space make the apparently simple questions of where to look for and how to then find the materials we need a grand challenge to contemporary physical science. This talk will emphasise that discovery synthesis of new inorganic materials is at the extreme forefront of this endeavour.
Since 2017, it has been clear that computational prediction can usefully direct the search for new structure types [1]. In the context of discovery, it is helpful to consider both appropriate definition of the word “new” and also how the claimed numbers of materials and the speeds with which they are accessed fit with the size of the space investigated, and the differences in how composition can vary between solids and molecules.
In this presentation, I will address the role of digital and robotic tools in discovery from the perspective of the experimental realisation of new materials with structures that differ from those in the databases in a manner that has consequence for their functional performance. This will include the demonstration that it is now possible under clear assumptions to guarantee to predict the crystal structure of a material based solely on its composition [2], the role of machine learning from data in supporting decisions by experimental researchers [3], and the acceleration of inorganic materials discovery with robots [4].
The role of these digital tools in a modern integrated materials discovery workflow will be presented with an example of the discovery (i.e., the experimental realisation in the laboratory) of a quaternary inorganic solid that displays high lithium ion conductivity that arises from its new structure. This leads to a different perspective on how lithium ions can attain high mobility in solids (accepted for publication, 2024). Such perspectives may prove generally helpful in the design of the fast ion transporting materials that we will need across future energy technologies.
Gielgud-I1
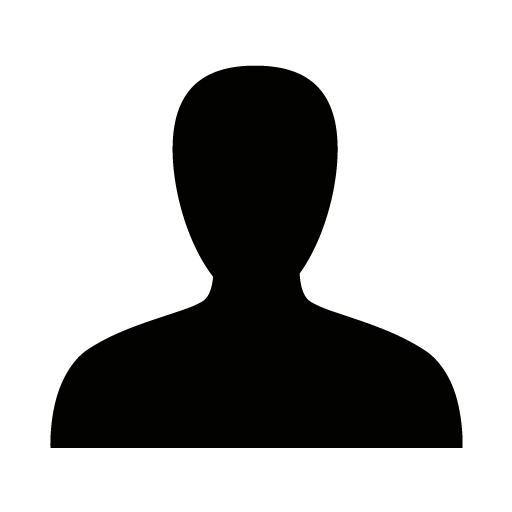
The ABO3-type perovskite oxides have wide applications in many fields such as solid oxide fuel cells and electrolyzers, oxygen storage materials, catalytic water splitting, and oxygen permeation membranes. In recent years, with the development of high-entropy ceramics, the flexibility of composition tailoring in ABO3 materials has attracted significant attention as an entropy regulation system. Compared with classical solid solution doping, high-entropy ABO3 can exhibit superior properties as solid oxide cell electrode materials including significantly improved high-temperature stability and better electrochemical performances.
The characteristic local structural distortion brought about by the significant increase in configurational entropy may have important effects on the ion transport behavior of ABO3-type materials, thereby further affecting the microstructural evolution during their high-temperature sintering process and the electrochemical properties involving ion conduction performance. In this report, we will introduce our recent work on the air electrode materials for solid oxide cells, namely high-entropy AMnO3 and ACoO3, examining the correlation between configurational entropy and related local structural distortions with the generation, distribution, and transport of oxygen and metal ion vacancies, and discussing the mechanisms influencing the sintering and electrochemical performance of the materials. Through such work, we hope to enhance the understanding of new structure-function relationships in entropy-regulated ABO3-type oxides, thereby promoting the applications of high-entropy oxide materials in solid oxide cells and other relevant devices requiring integrated structural-functional properties.
Shi, Y.; Ni, N.; Ding, Qi.; Zhao, X. Tailoring high-temperature stability and electrical conductivity of high entropy lanthanum manganite for solid oxide fuel cell cathodes, J. Mater. Chem. A 2022, 2256-2270
Shi, Y.; Zhang, H.; Ren, G.; Xiao, W.; Li, L.; Hu, Y.; Reddy, K. M;, Zhao, X.; Zhu, L.; Ni, N. Role of mixed entropy in sintering of manganite-based perovskite oxides: Faster densification with delayed grain growth. Acta Mater. 2024, 271, 119906.
Gielgud-O1
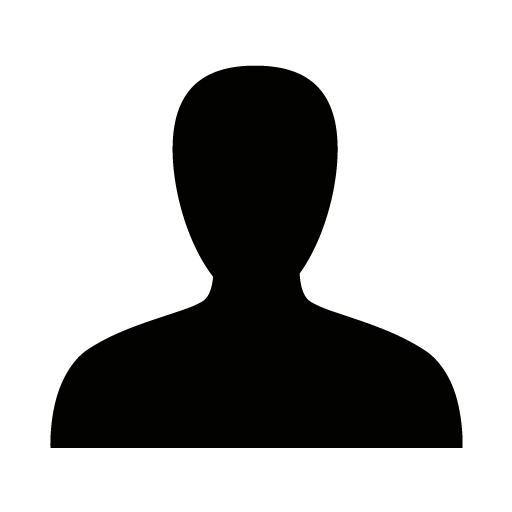
Solid-state batteries (SSBs) are attracting great interest leading to potentially higher energy and power densities compared conventional Li-ion batteries based on liquid electrolytes. However, they are plagued by the development of advanced solid electrolytes (SEs), mainly lacking in ionic conductivity and electrochemical stability; thus, the ongoing quest for exploration of new materials and compositions.
Inducing a large structural disorder, i.e. high configurational entropy, has recently emerged as a new strategy to overcome limitations of conventional SE materials. In this regard, few multicomponent SE materials have been reported up to now, showing favorable charge-transport properties [1,2]. However, a thorough understanding on how configurational entropy (ΔSconf) affects ionic conductivity is lacking. Here, we successfully synthesized a solid solution series of halogen-rich lithium argyrodites with the general formula Li5.5PS4.5ClxBr1.5−x (0 ≤ x ≤ 1.5) [3]. Using neutron powder diffraction and 31P magic-angle spinning nuclear magnetic resonance spectroscopy, the shared S2−/Cl−/Br− occupancy on the anion sublattice was quantitatively analyzed. We show that anion disorder positively affects Li-ion dynamics, leading to a room-temperature ionic conductivity of 22.7 mS cm−1 (9.6 mS cm−1 in cold-pressed state) for Li5.5PS4.5Cl0.8Br0.7 (ΔSconf = 1.98R). To the best of our knowledge, this is the first experimental evidence that configurational entropy of the anion sublattice correlates with ion mobility. Our results indicate the possibility of improving ionic conductivity in ceramic ion conductors by tailoring the degree of compositional complexity. Moreover, the Li5.5PS4.5Cl0.8Br0.7 SE allowed for stable cycling of single-crystal LiNi0.9Co0.06Mn0.04O2 (s-NCM90) composite cathodes in SSB cells, emphasizing that dual-substituted lithium argyrodites hold great promise in enabling high-performance electrochemical energy storage.
Fleming-I1
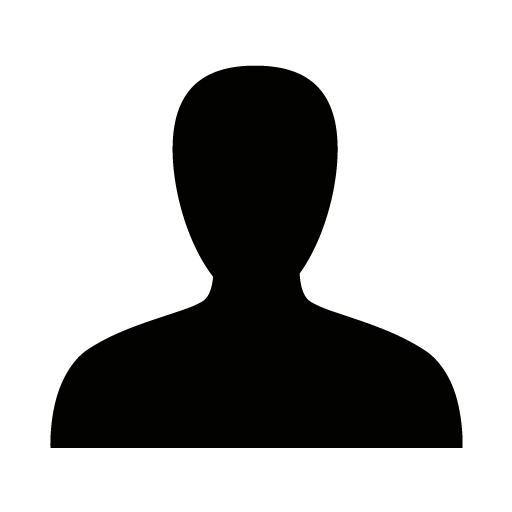
Li-ion batteries contain an ensemble of micron-sized redox-active particles. Understanding lithium reaction and transport rates in individual battery particles are essential for resolving the fundamental lithium insertion process and for accurate modelling and design of battery devices. Unfortunately, it is very difficult to probe the behavior in individual battery particles using electrochemical measurements conducted on porous electrodes. In this presentation, we use the microelectrode array to enable the electrochemical charge and discharge of individual NMC battery particles. By characterizing a statistically significant number of particles, we show that the smaller particles are no faster than the larger particles [1]. This result is in stark contradiction to conventional battery transport models, and is a result of electrolyte penetration into the polycrystalline secondary particles which were previously not considered in these models. We further show that single-crystal particles have slow reaction and diffusion times even in single particles, a result of the absence of cracks. These results highlight promises for microelectrodes to uncover previously hidden transport mechanisms in energy storage materials.
Fleming-O1
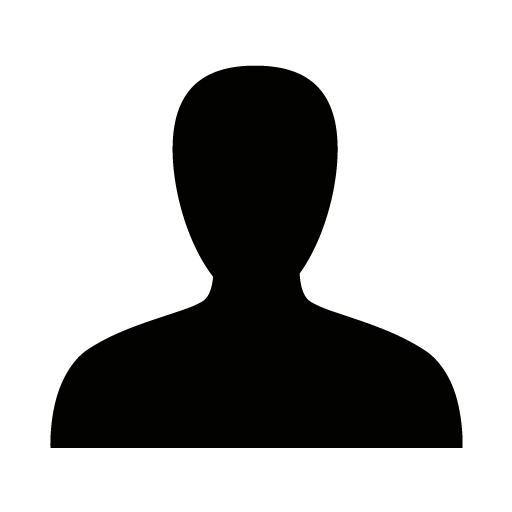
Solid state batteries (SSBs) are one of the emerging options for next-generation energy storage systems due to their improved safety and high power/energy densities. In composite SSB electrodes, many active material (AM) particles are randomly and three-dimensionally distributed together with solid electrolyte (SE) particles, forming complex ion and electron conduction pathways. Such complicated mass transport pathways lead to a local depletion of ion and/or electron suppy in the electrode, especially at high current densities, resulting in a three-dimensional (3D) heterogeneous electrochemical reaction within the AM particle ensemble and within each individual AM particle. Such heterogeneous reactions can degrade the capacity/power output and lifetime of SSBs. Therefore, an understanding of the influence of heterogeneous reactions on battery performance is important for the development of high-performance SSBs. However, there are few techniques that allow tracking of 3D heterogeneous reactions within an AM particle ensemble in a composite SSB electrode with sufficiently high spatial and temporal resolution.
Here, we developed an imaging technique that allows 3D operando tracking of the evolution of the heterogeneous reaction within the AM particle ensemble in the electrode and each individual particle in the ensemble with sufficiently high spatial (~200 nm) and temporal (40 min.) resolution, based on our previously reported technique using computed-tomography with X-ray absorption fine structure spectroscopy (CT-XAFS) [1], and investigated the heterogeneous reaction in composite SSB electrodes. Furthermore, we quantitatively analyzed the optimal particle parameters (e.g., particle size, shape) based on the data set of both chemical and geometric information of over 107 voxels and 102~103 of AM particles obtained by the 3D imaging.
Composite SSB cathodes were prepared by mixing octahedral single-crystal Li(Ni1/3Mn1/3Co1/3)O2 (NMC) particles with an average size of ~ 3 or 10 µm and Li2.2C0.8B0.2O3 (LCBO) solid electrolyte in a weight ratio of 5:5. A model SSB was then fabricated using this composite cathode, Li foil as the anode, and LCBO as the solid electrolyte. We performed the imaging nano CT-XAFS measurements near the Ni-K edge, employing an imaging optics consisting of a condenser zone plate and an objective Fresnel zone plate. The charge state distribution in the electrode was evaluated from the peak top energy of the Ni-K edge at each voxel in the obtained 3D image.
We three-dimensionally observed the evolution of the electrochemical reaction in 102~103 NMC particles in the region of 50×50×50 µm3 in the composite SSB electrode during constant-current electrochemical of 0.05~0.3C. The electrochemical reaction proceeded heterogeneously both in the whole AM particle ensemble and in each single particle. We statistically analyzed the relationship between the features of the electrochemical reaction in each particle such as average state-of-charge (SOC), intra-particle reaction inhomogeneity, and inter-particle reaction inhomogeneity, and particle parameters (e.g. spatial coordinates, volume, and shape). In the investigated electrode, larger particles tended to react heterogeneously within each particle while smaller particles tended to react heterogeneously between each particle, thus there was an optimal AM particle size that allowed for a uniform reaction. Our technique allows for the quantitative determination of the optimal active material parameters through the data-driven analysis of the experimentally obtained information of the heterogeneous reaction in the AM particle ensemble in a composite SSB electrode. Therefore, our technique provides useful information for SSB electrode design that cannot be obtained by other existing techniques.
Fleming-O2
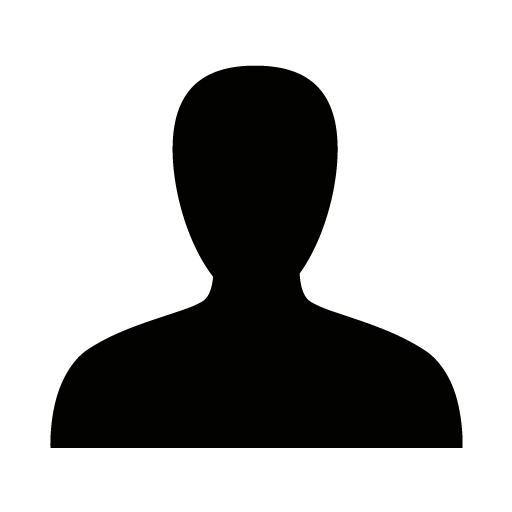
Intensive research is being conducted to further improve the performance of lithium-ion secondary batteries. Conventional positive electrode materials exhibit excellent charge-discharge cycle characteristics because only Li-ions are extracted and inserted while maintaining the crystalline structure of the material. In contrast, Li-rich layered oxide (LLO) materials with a low-crystalline structure achieve high energy density and extraction/insertion of a large amount of Li-ions. In this study, X-ray total scattering measurements were performed at the high-energy X-ray diffraction beamline BL04B2 at the SPring-8 synchrotron radiation facility (Sayo, Japan). The crystal structure variations during the charging and discharging of LLO were evaluated. The structural parameters in the various states of charge/discharge of LLO material 0.4Li2MnO3−0.6LiNi0.5Mn0.5O2 were determined through a crystal pair distribution function-based structural refinements using experimental G(r). Crystal pair distribution function analysis reveals that the constituent transition metals migrate substantially due to delithiation in the initial irreversible charging. We clarified the existence of a low-crystal structure in LLO formed by the crystal structure change accompanying the migration of pillar metal ions that support the Li-deficient layer during the extraction of Li-ions [1].
Westminster-I1
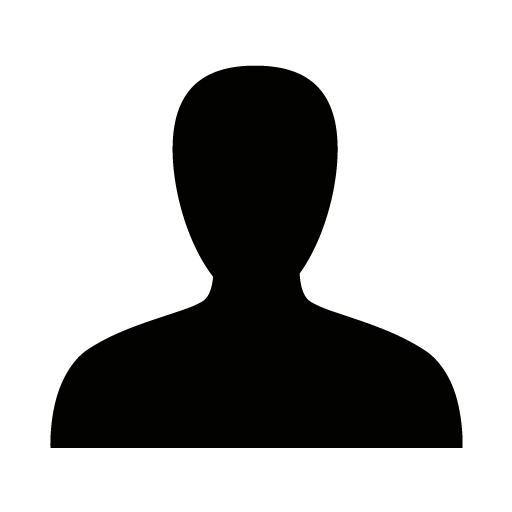
Oxide-ion conductors and proton conductors have attracted considerable attention because of their potential applications such as fuel cells, and gas sensors. Exploring novel ionic conductors with high ionic conductivity is an important but challenging task. Our group tackled this task by several strategies, and successfully discovered novel ionic conductors. We also investigate ion migration path and mechanism through the precise structure analysis.
Although Dion−Jacobson phases have been investigated in various fields, there are no reports on oxide-ion conduction. Through the screening by the bond valence method, we have found that Dion−Jacobson phase CsBi2Ti2NbO10−δ exhibits high oxide-ion conductivity.[1] From the high temperature neutron diffraction study, we revealed that the high conductivity is attributable to the large anisotropic thermal motions of oxygen atoms, the presence of oxygen vacancies, and the formation of oxide-ion conducting layers in the crystal structure. Ba3Y2O5Cl2 is discovered as the first examples of oxide-ion conducting oxychlorides.[2] Ruddlesden–Popper (RP) oxychlorides were expected to show high oxide-ion conductivity, because they have larger free and lattice volumes than RP oxides, leading to low activation energies. Two dimensional oxide-ion migration in Ba3Y2O5Cl2 is indicated from the DFT calculations. By investigating Ba7Nb4–xMo1+xO20+x/2 materials, we found that Ba7Nb3.8Mo1.2O20.1 exhibits high oxide-ion and proton conductivity.[3] Total direct current conductivity at 400 °C in wet air of Ba7Nb3.8Mo1.2O20.1 was 13 times higher than that of Ba7Nb4MoO20. Ab initio molecular dynamics (AIMD) simulations, neutron-diffraction experiments at 800 °C, and neutron scattering length density analyses of Ba7Nb3.8Mo1.2O20.1 indicated that the excess oxygen atoms are incorporated by the formation of both 5-fold coordinated (Nb/Mo)O5 monomer and its (Nb/Mo)2O9 dimer with a corner-sharing oxygen atom and that the breaking and reforming of the dimers lead to the high oxide-ion conduction. Ba2LuAlO5 exhibits high proton conductivities, high diffusivity and high chemical stability without chemical doping.[4] Ba2LuAlO5 is a hexagonal perovskite-related oxide with highly oxygen-deficient hexagonal close-packed h′ layers, which enables a large amount of water uptake x = 0.50 in Ba2LuAlO5·x H2O. Ba7Nb4MoO20 is one of the promising ionic conductors. However, the chemical order/disorder of Mo and Nb atoms was not revealed because Mo and Nb have similar scattering power for both X-ray and neutron. Therefore, we have combined resonant X-ray diffraction, solid-state nuclear magnetic resonance (NMR) and first-principle calculations to reveal the chemical order in Ba7Nb4MoO20.[5] NMR provided direct evidence that Mo atoms occupy only the M2 site near the intrinsically oxygen-deficient ion-conducting layer. Resonant X-ray diffraction determined the occupancy factors of Mo atoms at the M2 and other sites to be 0.50 and 0.00, respectively.
Westminster-O1
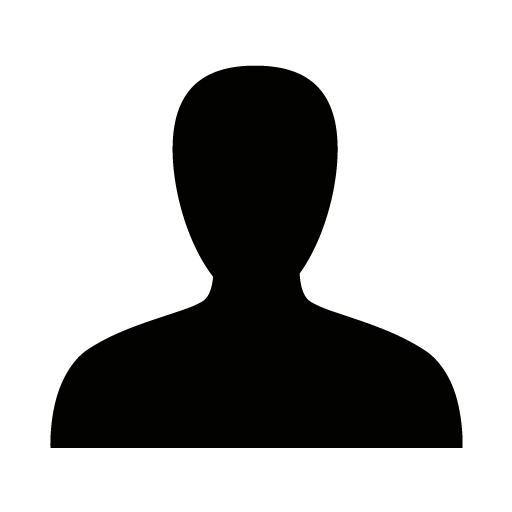
A relevant point in circular economy is the possibility to recycle wastes in a so-called “waste to treasure” attitude. For this approach to be economically sustainable, the purification and separation steps should be minimized, thus requiring robust catalysts. Double perovskite samples of composition Sr2Mg1-xMnxMoO6-δ (SMMO) have been considered because of their versatility towards different feeding gasses and resistance towards sulfur impurities, opening to the possible use of these catalysts with direct waste-derived fuels. [1] Catalysts characterized by different amount of Mg/Mn have been prepared (with x = 0, 0.2, 0.5 and 1) and studied as both catalysts for the Reverse Water Gas Shift (RWGS) reaction – relevant for optimization of H2 content in syngas for E-fuels synthesis – and anode materials in Solid Oxide Fuel Cells (SOFC) – with direct utilization of the fuel thanks to the high operating temperature. The synthesis was carried out via the Pechini route, using a previously optimized quantity of ethylene glycole (EG/CA = 3). X-Ray Diffraction (XRD) has shown the formation of the desired phase, with only minor impurities of MgO and SrMoO4, that could lower the overall performance of the cell. [2,3] Scanning Electron Microscopy (SEM) images have shown polyhedric-like shape, principally due to the high calcination temperature. The thermo-catalytic tests, performed using a gas blend of 80% H2 and 20% CO2, have shown a “twins”-like behaviour of the samples, also supported by the H2-TPR analysis (x = 0 is similar to x = 0.5, while x = 0.2 is similar to x = 1), hypothesising the absence of Mn (II) in the x = 0.5 sample; this results in the presence of Mn (III), known in previous work to possess a good activity toward oxygen exchange that, together with the Lewis basicity of the Mg (II) cation favours the coordination of CO2 and, consequently, its reduction. [4,5] The same gas blend was used to evaluate the electrochemical behaviour of the SMMO materials as SOFC anode by means of the Electrochemical Impedance Spectroscopy (EIS) technique, comparing the results to a benchmark test in H2 only as fuel. Interesting outcomes have been achieved in complete cells’ tests, using different feeding mixtures. SMMO materials demonstrate to be sustainable, versatile and robust catalyst – and electrocatalysts. The detailed characterization of the electrode powder, and the comparison between catalytic and electro-catalytic behaviour allows to go deeply into the fuel oxidation mechanism and to relate composition, structure and properties.
Westminster-O2
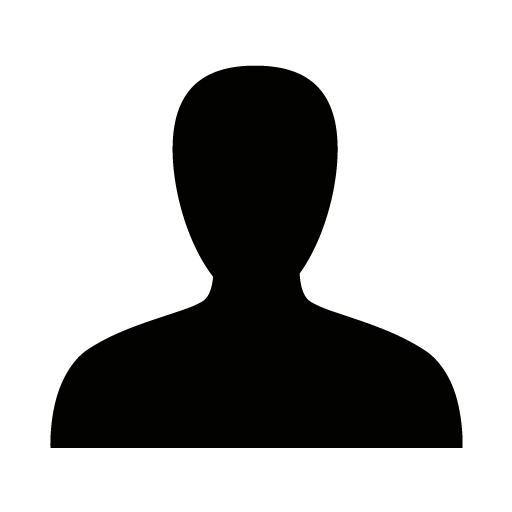
Dense membranes made of mixed ionic and electronic conducting oxides have been widely applied for high-purity O2 production[1] and partial oxidation of CH4[2], as well as water splitting[3]. As one of the most promising membrane materials, doped alkaline earth titanates, e.g. SrTiO3 doped by Mg or Fe (≤ 25 mol%) at Ti site, showcase great advantages in chemical stability under reducing conditions over the barium/lanthanum strontium cobalt ferrite perovskite-type oxides[4, 5]. However, a conductivity minimum, caused by a depletion of charge carriers at intermediate oxygen partial pressures (~10-8-10-6 bar), may limit the performance of the doped alkaline earth titanates in application scenarios such as water splitting. One solution proposed here is to introduce additional dopants that sustain a mixed valence state for charge transfer over a broad range of oxygen partial pressure (Po2). In addition, the thickness reduction of membrane components also represents a state-of-the-art strategy for performance promotion due to the physically shortened transport passes[6]. The current work has explored and validated both of the two strategies based on characterizations of crystal structure, microstructure, and conductivity as well as oxygen permeation.
The doping strategy has been applied for CaTiO3 as an example. The Ti site is doped by Sc with a stable valence of 3+ together with Cr or Ru with variable valence states. The Sc dopant can introduce mobile charge carriers (oxygen vacancies) supporting ionic conduction[7], while the Cr or Ru dopant are expected to provide charge carriers that are mobile and sustainable over a wide Po2 range guaranteeing a high electronic conductivity[8, 9]. However, the synthesized materials with dual dopants show lower total conductivities as compared with the materials with a single dopant, suggesting a cancel-out effect likely caused by the charge balancing between two dopants. Further efforts to alleviate such an effect can rely on the concentration optimisation of the two dopants.
The strategy towards the membrane component has been conducted for the Sr0.98Ti0.75Fe0.25O3-δ materials using commercially available powders. As the free-standing membrane becomes highly fragile during production and handling when the thickness is continuously decreased to ~100μm, it is necessary to apply a thicker but porous layer as the mechanical support for the dense thin membrane layer, forming a so-called asymmetric membrane. By employing a well-developed tape casting technique, a two-layer tape has been cast in sequence and co-sintered, obtaining the asymmetric membrane. The thickness of the membrane layer has been successfully decreased to ~20 μm, which effectively enhances the oxygen permeation suggesting a promising prospect for application in water splitting. A further development of catalyst is required to overcome the increased limitation from the surface exchange.
Westminster-O3
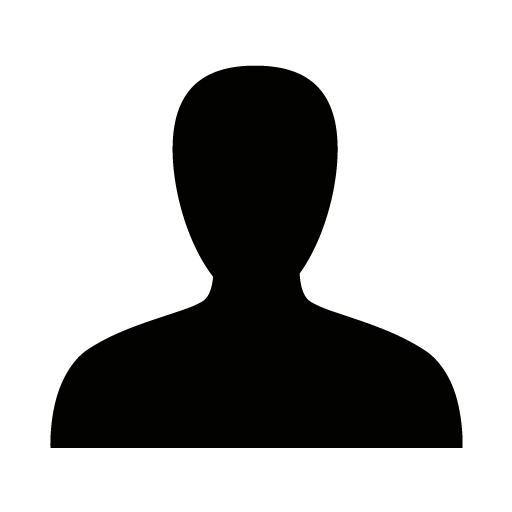
Most of Solid Oxide Fuel/Electrolyser Cells (SOFC/SOEC) air electrode materials, such as
La0.8Sr0.2MnO3 (LSM) or La0.6Sr0.4Co0.2Fe0.8O3-δ (LSCF), display the perovskite structure or related
structures. They are made of Rare Earth Elements (REE) on the A site whose partial substitution allows
to tune their transport properties.[1] However, REE have been listed as critical resource by the European
Union, mostly due to the concentration of the mining industries in China. In this context, the aim of the
NOUVEAU project, funded by the EU, is the development of novel electrode coatings and interconnect
for sustainable and reusable SOEC with the objective to reduce by at least 30 % the amount of REE
used in a SOEC stack. This implies to find new REE free materials, mainly for the air electrode.
Interestingly, recent papers showed good mixed ionic-electronic conductivity, promising oxygen
permeability, thermal expansion coefficients (TECs) compatible with typical solid electrolytes and low
area specific resistance (ASR) values for Ca2Fe2O5 derivatives. [2-7] ASR of 0.22 Ω.cm2 at 750 °C with
a cerium gadolinium oxide (CGO) electrolyte and 0.23 Ω.cm2 at 700 °C with Sm0.2Ce0.8O1.9 (SDC)
electrolyte were reported for Ca2Fe1.3Mn0.7O5+δ and Ca2Fe1.8Co0.2O5+δ, respectively, the target for the
application being an ASR lower than 0.15 Ω cm2 at 700°C.[6-7]
These materials display the brownmillerite structural type which can be viewed as an oxygen deficient
perovskite with the nominal formula A2B2O5+δ or ABO2.5+δ. The oxygen vacancies ordering in the
brownmillerite structure results in alternating layers of corner-sharing BO6 octahedra and BO4
tetrahedra. The latter being oxygen deficient, consequently, oxygen diffusion along these chains is
expected while a good electronic conductivity can be obtained using transition metal ions with flexible
red-ox properties such as manganese, iron and cobalt on the B-site.
Based on the promising results reported in literature, di calcium iron oxides-based materials with
manganese or cobalt in the B site were synthesized via a citrate-nitrate route. First ASR measurements
were carried out by electrochemical impedance spectroscopy. An ASR of only 1.99 Ω cm2 at 800 °C
was measured for the most promising Ca2Fe0.5Mn1.5O6-δ material mainly because of a lack of ionic and
electrical conductivity. The ASR was considerably improved when the material was studied in
composite with CGO, reaching 0.60 Ω.cm2 at 800 °C. Moreover, the electronic conductivity was
improved by doping with Bi, Y or La on the Ca site. This led to an ASR of only 0.74 Ω.cm2 at 800 °C
for the pure Ca1.9Bi0.1Fe0.5Mn1.5O6-δ. Study of composite cathode is in progress.
A careful study of the electrochemical response of these systems will be presented.
Westminster-S1
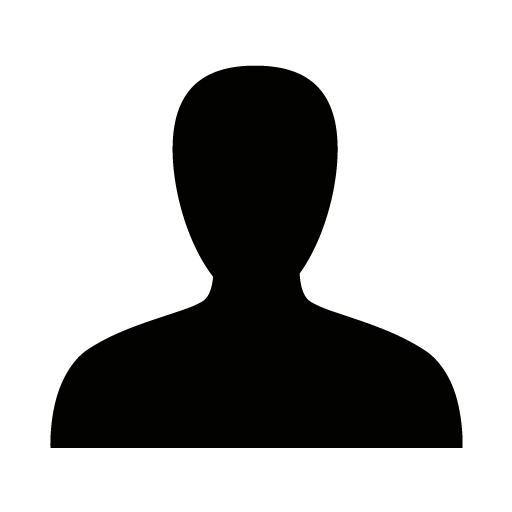
Bruker AXS provides scientists with a range of high-performance X-ray diffractometers to deliver the most detailed insight into the structural factors relevant to ion transport. This presentation will provide an introduction to information that can be obtained using various diffraction techniques and the instruments used to achieve them.
SC-XRD uniquely determines the precise atom coordinates required for understanding subtle structural details that affect ion transport such as polyhedral volume, bottleneck size, coordination site environments and anisotropic thermal motion of ions which indicate ion migration pathways. Bruker AXS provide a range of diffractometers running the APEX5 software suite to enable you to gain a deep understanding of the structure of solid state ionics quickly and conveniently on even the most challenging samples.
X-ray powder diffraction is widely used to study the long range structure of crystalline ion conductors, and conveniently provides accurate information on lattice volume and lattice symmetry. Rietveld refinement using TOPAS allows you to model atomic lattice coordinates. The speed and convenience of XRPD means the method is perfectly suited to measure samples under non-ambient conditions.
X-ray diffraction is also applied to the study of structural changes in assembled battery cells In operando diffraction allows researchers to follow the reactions that occur during cycling and to monitor degradation behavior to battery performance.
Short-range structure, such as local distortions, can greatly affect ion transport. Pair distribution function (PDF) data consists of both Bragg and diffuse scattering information. Therefore, PDF data probes not only long-range structures, such as lattice parameters, but also short range structures such as local distortion. PDF analysis can be performed on both single crystal and powder samples.
Reference:
Gao, Y. et al. Classical and Emerging Characterization Techniques for Investigation of Ion Transport Mechanisms in Crystalline Fast Ionic Conductors. Chemical Reviews 2020, 120, 13, 5954 – 6008.
St.James-I1
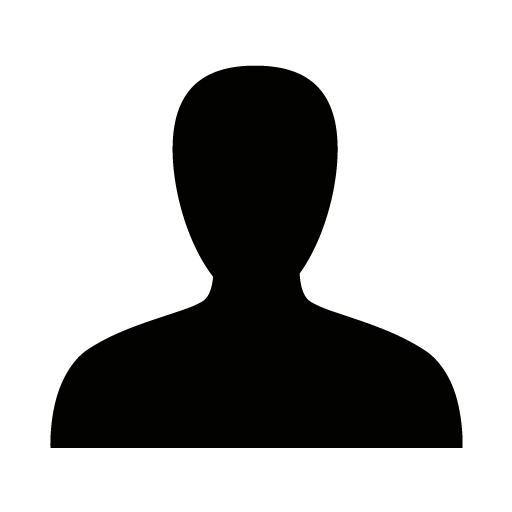
Protonic ceramic electrochemical cells (PCECs) have been developed as a promising technology for efficient power generation and hydrogen production via steam electrolysis. However, the current operating temperature range of 450-600°C still limits their widespread application and adoption in ceramic electrochemical cell technology. To address this issue, lowering the operating temperature range to 250-450°C has been proposed, which would expand the range of materials for building PCEC systems and reduce their cost. In this presentation, I will discuss our group's series of approaches to redesign PCECs. These approaches include using readily fabricated single-grain thick, chemically homogeneous, and robust electrolytes, as well as newly developed positive electrode materials. Our innovations have simultaneously reduced the electrolyte ohmic resistance, electrolyte-positive electrode contact resistance, and electrode polarization resistance, while also improving durability. We have demonstrated that through the PCEC materials and architecture we have designed, PCECs can be employed for power generation and hydrogen production at 250-450°C, thereby transforming the architectures and removing previous operational constraints of ceramic electrochemical cells for scaled operation. PCECs can therefore be considered as one part of the growing hydrogen economy, while leveraging the existing fossil fuel infrastructure and achieving higher energy efficiency and lower emissions.
St.James-O1
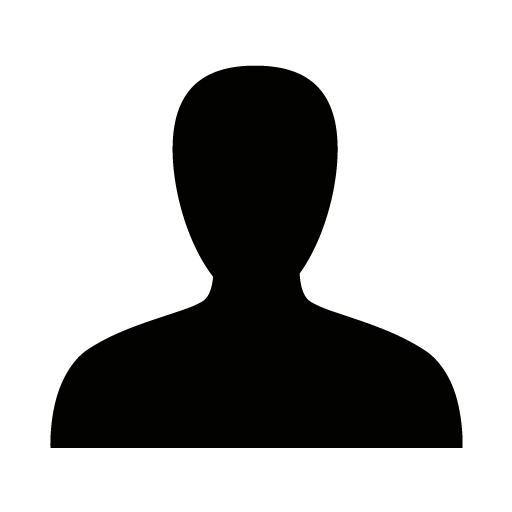
Protonic ceramic electrochemical cells (PCECs) are garnering considerable attention for their ability to efficiently and reversibly convert chemical fuels into electricity at temperatures below 600°C. However, the oxygen electrode's slow reaction kinetics lead to inadequate reaction activity and reduced cell stability. Therefore, developing a highly active oxygen electrode is crucial for achieving high-performance PCECs. Introducing high-valence cations (such as V5+, Nb5+, and Ta5+) into the SrCoO3-𝛿 lattice has recently been identified as a potential strategy to enhance electrocatalytic activity and stability. Nonetheless, Sr-based oxygen electrodes face durability issues due to Sr segregation during PCEC operation. As an alternative, BaCoO3-𝛿 based materials are attracting attention as Sr-free oxygen electrodes, benefiting from the Ba cation's low electronegativity, large ionic radius, and cost-effectiveness. Inspired by previous studies, we designed Ta-doped BaCoO3-𝛿 perovskite oxides, which exhibit exceptional activity and durability for bifunctional oxygen electrodes. This work demonstrates the effectiveness of Ta doping in the BaCoO3-𝛿 lattice, which promotes the formation of a cubic perovskite structure and enhances electrocatalytic activity, leading to high-performance PCECs.
St.James-O2
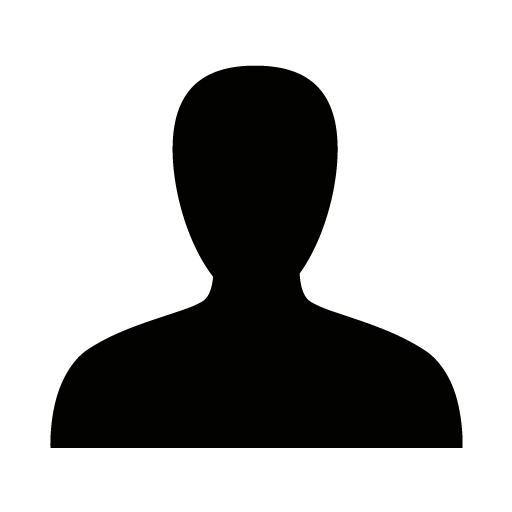
Oxygen electrode/electrolyte interface is critical to the performance and stability of solid oxide fuel cells. Typically, the formation of a tight oxygen electrode/electrolyte interface is achieved by minimizing the surface energy between solid phases during high temperature sintering. For conventional LSM and LSCF electrodes, a large number of studies have shown that the formation of LSM/YSZ and LSCF/GDC interfaces is accompanied by cationic interdiffusion, which may subsequently lead to phase segregation, interfacial reactions, and performance degradation under operating conditions. However, researchers have paid little attention to the oxygen electrode/electrolyte interface of protonic ceramic fuel cells (PCFCs), and the formation and evolution mechanism of the interface under practical working conditions are still unclear. Herein, LSM, LSF, PBSCF, and BCFZY oxygen electrodes were prepared on BaZr0.1Ce0.7Y0.1Yb0.1O3-δ electrolyte by high-temperature sintering and treated under actual working conditions. The effects of electrode composition, treatment conditions on ionic interdiffusion and elemental segregation were systematically investigated by exploring the morphology, phase composition, elemental distribution and valence states on the electrolyte surface, and the effects on the oxygen electrode/electrolyte interface were further investigated.
Moore-I1
Graduated in Materials Science at Instituto Sabato, UNSAM, Argentina
PhD Technical University Berlin, Germany
Since 2018, staff researcher at the Helmholtz-Zentrum Berlin (HZB) in the Interface Design Department of the Energy Materials In-situ Laboratory (EMIL), currently coordinating HZB’s characterization strategies in the Care-O-Sene and Green-QUEST projects. and the the HZB Photon school held yearly at BESSY II, offering lectures and practical trainings at several beamlines.
Research focus on in-situ and operando investigations of energy materials and devices, such as catalysts and solid oxide cells. Complementary synchrotron-based spectroscopies, scattering and imaging methods are combined to investigate energy conversion processes.
The performance of solid oxide cells (SOCs) is highly influenced by the electrocatalytic activity of the working electrode material at the solid/gas interface. New generations of working electrode materials are usually highly functionalized oxides and cermets of complex stoichiometries, designed to perform as mixed ionic/electronic conductors for efficient operation as anode or cathode at intermediate temperatures (500 – 700 °C)[1]. Examples of these materials include doped Sr(Ti,Fe)O3 perovskites and co-doped CeO2 with alloyed with 3d metals. Both undergo complex transformations, specifically surface modifications such as nanoparticle ex-solution and alloying upon reduction to boost electrocatalytic activity [2].
Here we present how to gain insights into the role of each element while by using highly brilliant synchrotron light for element specific techniques such as time-temperature resolved near ambient pressure X-ray photoelectron and absorption spectroscopies (NAP-XPS/XAS) to probe the (near) surface chemistry, oxidation states and elemental segregation at or near the solid/gas interface. Grazing incidence X-ray diffraction provides a complementary structural perspective. In a next step we present device-driven optimization using model cells to apply polarization and other electrochemical techniques such as voltammetry, chronoamperometry and electrochemical impedance spectroscopy along with the synchrotron-based techniques. Polarization proved to be an important variable capable of completely modifying the surface chemistry of the working electrode, offering opportunities for tuning the surface chemistry or regenerating the working electrode material by reversing exsolution, remove carbon deposits or investigate SOCs in fuel or electrolysis (SOFC/SOEC) operation modes.
We include three study cases on yttria-stabilized zirconia electrolyte supported model cells: The first uses a Ni-doped Sr(Ti,Fe)O3 perovskites as working electrode for bimetallic Ni-Fe exsolution and reversible SOFC/SOEC operation in H2/O2 gases [3]. The second uses a Ni-Co co-doped Sr(Ti,Fe)O3 electrode for trimetallic exsolution of Ni-Co-Fe nanoparticles and SOFC operation with the C1 fuel CH4 [4]. The third case is a cermet, co-doped CeO2 with a Ni-Fe alloy, for CO2/CO/H2 co-electrolysis in SOEC mode [5,6].
Moore-O1
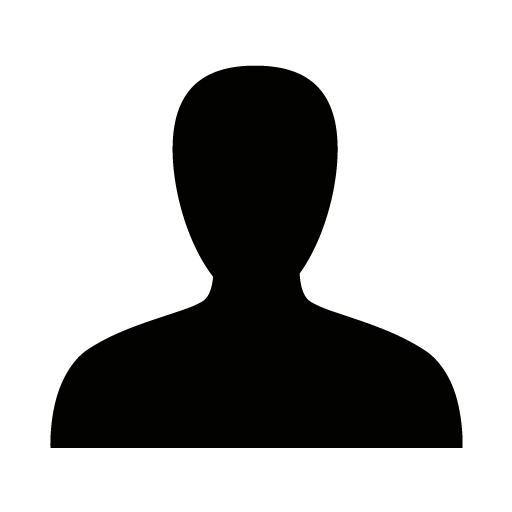
The change of the oxygen content in a material using an electrochemical cell is an important asset to understand the variation of the Fermi energy in materials, paving the way for Fermi level engineering as a versatile toolkit for designing a broad range of materials according to specific applications. The electrochemical cell is constructed by top and bottom electrodes deposited on an oxygen ion conducting electrolyte. The reduction or increase of the oxygen content in the electrodes by cathodic or anodic polarization, is accompanied by an increase or lowering of the Fermi energy, respectively. The latter can be monitored together with chemical changes of the sample if the electrochemical cell is operated in an X-ray photoelectron spectrometer. We will present experiments using Y-stabilized zirconia, Fe-doped SrTiO3, or (anti-)ferroelectric perovskites as electrolytes, and (Sn-doped) In2O3, SrFeO3-δ and Sr-doped LaCoO3-δ as electrode materials. The use of the setup to quantify the Fermi energies of charge transition levels in either the electrolyte or the electrode will be demonstrated.
Moore-O2
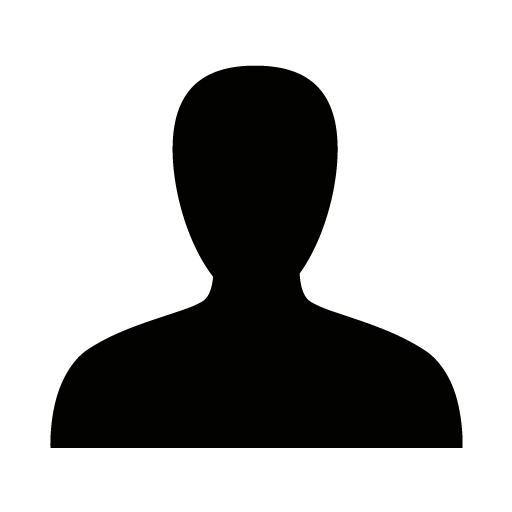
Although solid oxide fuel and electrolysis cells are already commercially available, the exact surface chemistry and reaction mechanisms for oxygen exchange at the air and fuel side are still only partly understood. It was realised that slight alterations of the surface chemistry during operation can have substantial impact on the electrochemical activity, which explains the tremendous scatter in the measured electrochemical activity of a given material1. In-situ ambient pressure XPS measurements on electrochemical model cells reveal the surface chemistry during electrochemical operation and its changes with time2, atmosphere and electrochemical polarisation3–5 and provide a direct link between surface chemical state and electrochemical activity. This talk presents a survey of own experiments that show the complex interplay of operation conditions, surface chemistry and electro-catalytic activity in oxygen and fuel atmospheres.
Specifically, this talk will present advanced designs for two and three electrode model cells6 that are optimised for in-situ characterisation in ambient pressure XPS as well as UHV7 and corresponding results. In oxidising conditions, primarily degrading factors were observed. Interestingly, the primary source of the fast initial degradation is not A-site cation segregation per se, but the formation of a SO42- adsorbate layer from sulphur trace impurities on various different materials1. In reducing conditions, mixed oxidation states of Fe2+/Fe3+ in Fe containing perovskites and Ce3+/Ce4+ in doped ceria4 are observable, and most likely the mediators for charge transfer to hydroxide4 or carbonate3,8 reaction intermediates in the rate determining step. Interestingly, only ceria exhibits a strongly enhanced Ce3+ fraction at the surface, whereas the Fe2+ on perovskite-type SrTi0.6Fe0.4O3-δ and La0.6Sr0.4FeO3-δ is not enriched at the surface. Lastly, cathodic polarisation in a reducing atmosphere can trigger the exsolution of catalytically active Fe0 metal particles9–11, on which the H2 release rate can be greatly enhanced, compared to the bare perovskite.
Moore-O3
The growing scale of renewable electricity sources (i.e., solar/wind energies) underscores the necessity for electricity storage to balance grid supply/demand across various time scales, which is critically required for the widespread integration of renewables into our energy infrastructure. One of the promising next-generation energy devices, solid oxide cells (SOCs) have been highlighted as a means of storing excess electricity as chemical energy. SOCs offer several advantages, including:(1) High efficiency combined with utilizing waste heat sources (e.g., geothermal energy/nuclear energy), (2) Flexible operations in electrolysis/fuel cell modes, and (3) Selective chemical product generation (e.g., CO/NH3/C2H6). Despite ongoing efforts in the commercialization of SOC technology, it continues to face hindrances primarily associated with degradation-related issues.[1] Notably, the UK government has set an ambitious long-term stability goal of over 87,500 hours (equivalent to 10 years) for SOCs by 2050.[2] Therefore, the “long-term durability” of the SOCs under operating conditions will be crucial for achieving the ambitious goals set for SOC.
The primary objective of this research is to comprehend the intricate structure and chemistry of interfaces in SOCs by systematically characterising the evolution from the pristine state to the postmortem state of aged SOCs. The investigation has specifically focused on internal interfaces, such as grain boundaries, establishing connections between the findings and the microscopic outcomes of the interfaces. A range of advanced characterization tools, including scanning electron microscopy (SEM), scanning transmission electron microscopy (STEM), low energy ion scattering (LEIS), secondary ion mass spectrometry (SIMS), and atom probe tomography (APT), has been employed to establish correlations among interfacial changes. The investigation spans a range of length scales, from scrutinizing interactions at individual grain boundaries (APT) to assessing areas exceeding 400 mm² (SIMS). The advanced characterisation techniques play a critical role in better understanding the degradation mechanism of SOC and achieving in-depth knowledge, providing insight to overcome durability issues.
Abbey-I1
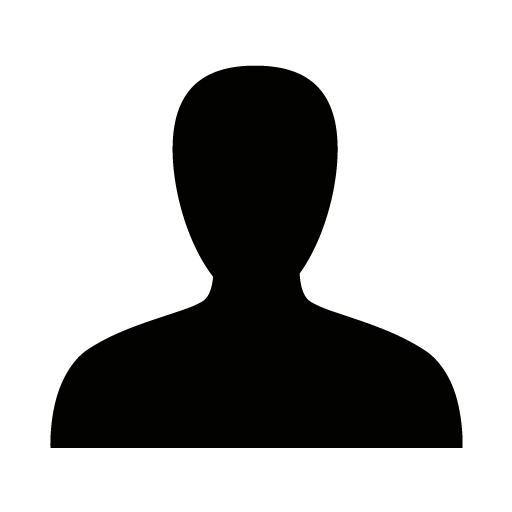
A-site layered double perovskites, derived from the parent material PrBaMn2O5+δ, have demonstrated exceptional suitability as electrode materials in solid oxide fuel cells (SOFCs). By introducing catalytically active metal cations onto the B-site of a single perovskite oxide, it becomes feasible to induce the exsolution of metal nanoparticles on the surface through a phase transition to the double perovskite structure under reducing conditions. Palladium, renowned for its outstanding catalytic activity, has been utilized in various applications, leveraging exsolution as a means to employ noble metals in minimal quantities. In this study, we scrutinize the phase segregation behavior and ionic environment of palladium incorporated on the B-site of Pr0.5Ba0.5MnO3-δ. The substitution of palladium in the manganese sites induces the creation of oxygen vacancies to accommodate square planar Pd2+. Upon undergoing reduction treatment, a phase transformation occurs, resulting in the formation of the A-site layered double perovskite, PrBaMn1.9Pd0.1O5+δ (L-PBMPd), accompanied by the exsolution of monometallic and bimetallic nanoparticles on its surface. Notably, this study reports, for the first time, the exsolution of metallic manganese in the form of an alloy nanoparticle with a composition of Pd3Mn. The achieved maximum power densities of 0.59 W cm-2 at 800 °C and 0.80 W cm-2 at 850 °C underscore the high performance of L-PBMPd as an anode material in SOFCs. This outstanding performance suggests potential synergistic effects of the Pd3Mn nanoparticle in fuel oxidation, positioning it as a promising alloy for catalytic applications.
Abbey-O1
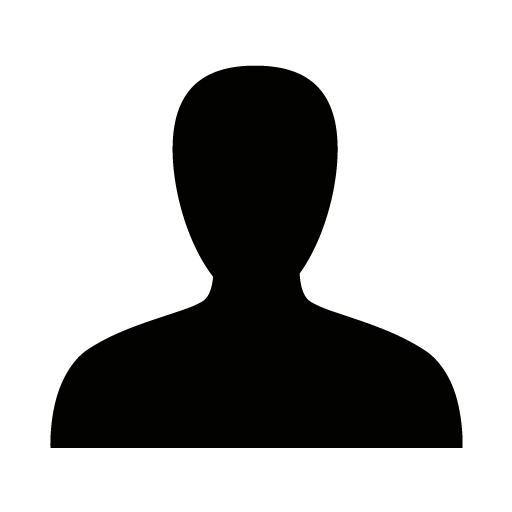
Metal nanoparticles on oxide surfaces hold considerable potential for application in heterogeneous catalysis and in electrocatalysis, particularly in the context of solid-state electrochemical devices such as fuel flexible fuel cells, electrolysers, and electrochemical membrane reactors. Exsolution is a process to achieve this modification of the solid support. It offers important advantages compared to conventional deposition of nanoparticles, the most prominent being the reduced tendency of the nanoparticles to agglomerate. Some insights into the structure, stoichiometry and functionality of individual material systems suitable for exsolution have already been gained, however, there is still a lack of knowledge in defect chemistry and the kinetics of exsolution.
From its size Ni2+ is expected to reside on the B-site positions of the perovskite structure of SrTiO3. However, B-site diffusion is known to be relatively slow because of the stable Ti-O-skeleton of the material which seems contradictory to the much faster exsolution process. Here, an interstitial diffusion mechanism for nickel and its dependency on different defects is investigated by density-functional theory (DFT) calculations as well as transient thermogravimetry.
Exsolution involves reduction of Ni2+ and the complementary oxidation of O2- which leaves the sample, resulting in a mass loss which can be tracked over time. With nickel diffusion being expected to be the dominant process diffusion information can be extracted from fitting this data. The studies are performed with systematic variation of several parameters, including the temperature, amount of nickel, amount of A-site and oxygen vacancies, and the particle sizes of the sample.
This approach aims to develop a more generalized understanding of the kinetics of exsolution, with the overarching goal of facilitating the tailored design of materials according to their applications.
Abbey-O2
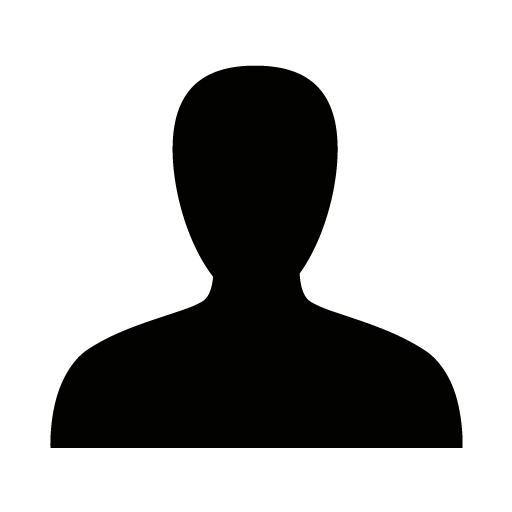
Since its inception a decade ago, exsolution has proven to be a powerful tool for the design of advanced energy materials. Therefore, the ability to tailor the size, morphology, and composition of the exsolved nanoparticles is critical to implement this technique to further improve the performance of such nanostructured catalysts [1]. Previously, ex-situ techniques had been used to probe this rather complex system, but the growing interest to understand the exsolution processes and the material changes during catalytic operation has paved way for the development of in-situ methods such as XRD, AP-XPS, and TEM [2]. So far however, poor information could be gathered with respect the nanoparticle size evolution during the exsolution process.
In this work, the exsolution of nano alloy with Ru-Ni composition from an A-site deficient double perovskite of the type (La2−xNiRuO6−δ, x = 0.15) [3], was investigated via in-situ X-ray scattering methods. In particular, the exsolution process was studied at 450 and 550°C for 5 h both by in situ XRD and small-angle X-ray scattering (SAXS). This latter method represents a novel approach and offers a direct and highly statistically relevant investigation of the size evolution of exsolved nanoparticles. The analysis of the SAXS) curves gave an insight into the formation of both surface and sub-surface nanoparticles.At 450 °C the particles formed on the surface increased from 0.5 to 2 nm, whereas the buried component grew from 3 to 10 nm in diameter. which agreed with the particle size distribution seen in the TEM images [3]. During the exsolution process over 5 hours we could monitor different nucleation events which corresponded well to the structural changes of the perovskite matrix and the reduction of the metal species monitored by in situ XRD and XANES spectroscopy, respectively.
Abbey-O3
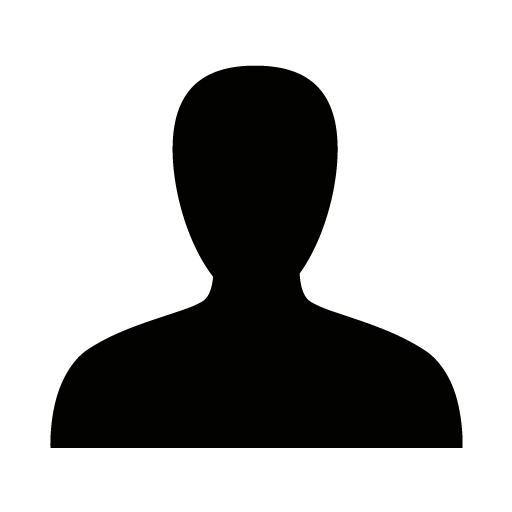
The development of nanostructured composite materials is critical for improving the efficiency of a variety of heterogeneous catalytic processes. Metal exsolution is a promising synthesis route for the formation of oxide-supported metal catalysts, allowing for the formation of well dispersed and highly active metal nanoparticles. While exsolution is often proposed as a route for enhancing particle stability (thus leading to catalytic systems with increased efficiency and extended lifetimes), recent work has shown that there are still limitations to the thermal stability of exsolved nanoparticles. Provided this, a fundamental understanding of the factors which impact the stability of exsolved nanoparticles remains essential to the development of optimized catalytic systems. In this work, the dynamics of exsolved Ni nanoparticles are investigated using an environmental STEM equipped with a secondary electron detector, providing a route for atomic-resolution characterization of the sample surface during and after the exsolution process. An epitaxially grown Ni-doped strontium titanate thin film is utilized for in situ experiments, which has a unique nanostructure that consists of a Ni-doped strontium titanate matrix with embedded, heteroepitaxial NiO nanocolumns. Exsolution from this material is characterized, providing evidence that two distinct populations of Ni nanoparticles form – those that precipitate above second-phase nanocolumns, and those which nucleate above the matrix. The dynamics of the two nanoparticle populations post-exsolution are highlighted and characterized by in situ STEM, with particular focus on the different behaviors of the two types in regards to Ostwald Ripening and particle migration.
Gielgud-I1
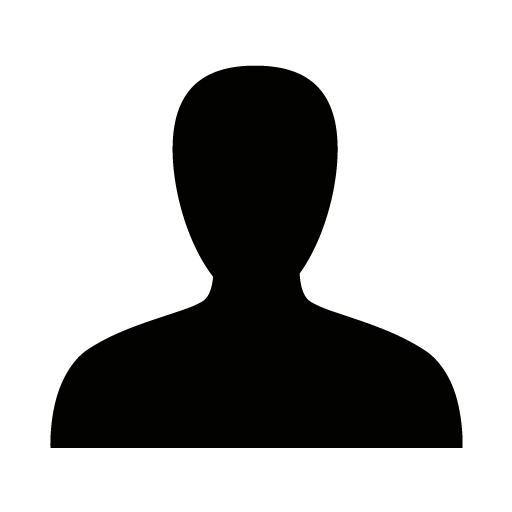
Conventional cathode materials of sodium-ion batteries (SIBs) are pre-sodiated and store Na-ions electrochemically. Interestingly, pre-potassiated materials can be appealing cathodes for SIBs. Potassium Prussian blue analogues (K-PBAs) is an excellent example. K-PBAs are cost effective and sustainable materials that are based on earth-abundant elements, and they have the structural remits to allow fast migration of large-sized ions. I will show in this talk that in a hybrid SIB cell, where Na+ is in the electrolyte and K+ is in the K-PBA cathode, not only is the electrochemical mechanism of cation intercalation in K-PBA dominated by K-ion rather than Na-ion, but the mechanism can be affected by the [Fe(CN)6]4- anion vacancies present in K-PBA. K-PBA with 25% anion vacancies exhibits two cation intercalation steps in a hybrid SIB cell, both being dominated by K-ion and displaying a ~0.2 V voltage increase compared with Na-PBA. In contrast, K-PBA with 7% anion vacancies exhibits both K-ion intercalation (>2.8 V vs. Na+/Na) and Na-ion intercalation (<2.8 V). This is because a higher vacancy level enhances K-ion diffusion in the PBA framework, which facilitates K-ion intercalation and suppresses Na-ion intercalation. A lower vacancy level deteriorates K-ion diffusion and thus enhances Na-ion intercalation. In a full-cell that is consisted of the K-PBA cathode and the hard carbon anode, the electrochemical process proceeds in a similar manner as the half-cells. K-ion dominates the intercalation in the K-PBA cathode while Na-ion dominates the intercalation in the hard carbon anode; therefore, a hybrid SIB is formed.
Gielgud-O1
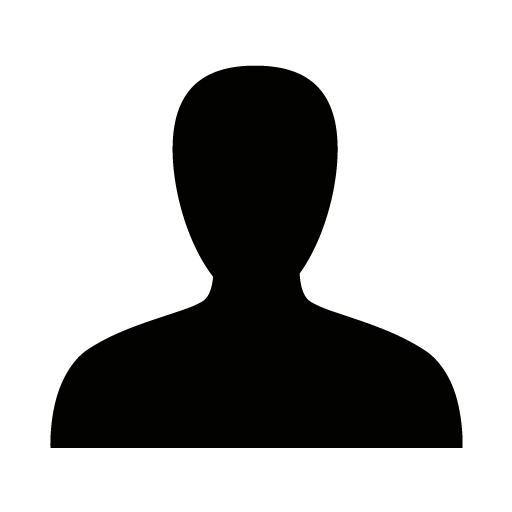
Heightened attention has been directed towards high entropy materials, owing to their distinct properties as potential battery cell components.[1] Lithium-doped high-entropy oxides (LiHEOs) exemplified by Li0.33(MgCoNiCuZn)0.67O exhibit notable lithium ion and electron conductivity.[2,3] This renders them promising as coating materials for NCM (Lithium-Nickel-Cobalt-Manganese Oxide) in conventional Li-ion battery (LIBs) cells, due to their high ionic and electronic conductivity. However, the stringent conditions and high temperatures required for their synthesis impose limitations on their practical application.
To overcome these challenges, a photonic curing strategy is proposed for the synthesis of LiHEO/HEO (high entropy oxide) materials. This approach successfully yields, for the first time, a nanoscale, homogeneous coating layer on NCM particles.[WO2023/280534 A1] The modified surface of NCM with LiHEO demonstrates exceptional electrochemical cycling stability, exhibiting a noteworthy 91% capacity retention at 1C after 350 cycles. This enhanced electrochemical performance can be attributed to the uniform coating, which effectively mitigates structural changes resulting from the interaction between the electrode active material and electrolyte.
In comparison to the uncoated NCM, the presence of a coating substantially reduces the formation of cracks in secondary particles. This reduction in crack formation prevents the electrolyte from further reacting with primary particles along the cracks. The findings suggest the potential of LiHEO-modified materials as a viable solution for improving the electrochemical performance and cycling stability of high nickel cathode active materials and thereby increasing the cycle life of LIBs. Further, photonic curing presents a novel synthesis and coating procedure for cathode active material particles that paves the way for surface modification of any heat sensitive material for a number of applications using a cost and energy efficient approach.
Gielgud-O2
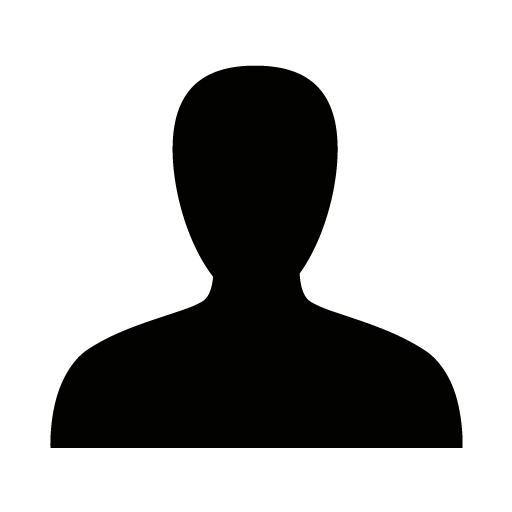
Solid-state batteries (SSBs) are a potentially safe, next-generation energy-storage technology [1-2]. For SSBs to be commercially viable, the development of solid electrolytes (SEs) with high ionic conductivity, high (electro)chemical stability, and good processability is imperative [2]. A novel approach to modifying materials, potentially leading to improved properties, is the high-entropy concept, characterized by a ΔSconf > 1.5R (where ΔSconf and R represent configurational entropy and ideal gas constant, respectively) [3]. However, the effect of configurational entropy on lithium transport remains largely elusive.
Recently, our group has extended the choice of high-entropy SEs to include lithium argyrodites by multianionic and/or -cationic substitution [4-6]. For multicationic substituted litihum argyrodites with the composition Li6.5[Ge0.25Si0.25Sb0.25P0.25]S5I, high ionic conductivity (> 10 mS/cm at 25 °C) and a low activation energy for conduction (0.20 eV) were observed, as shown by 7Li pulsed field gradient (PFG) nuclear magnetic resonance (NMR) spectroscopy and electrochemical impedance spectroscopy (EIS) [5-6]. These results were rationalized from a structural perspective via neutron powder diffraction combined with magic angle spinning (MAS) NMR spectroscopy. In this case, high S2‒/I‒ site disorder (up to ~11%) and lithium re-distribution led to shortened Li-Li jump distances, facilitating long-range ion diffusion [5-6]. Subsequently, we have explored a series of multicationic substituted lithium argyrodites by tuning the cationic stoichiometry. Through complementary EIS and 7Li PFG NMR measurements, we found that compositionally complex substitution helps to significantly improve Li-ion mobility. Notably, our study provides clear evidence for the correlation between configurational entropy and ion mobility and indicates the potential for enhancing conductivity in ceramic ion conductors by tailoring the compositional complexity, opening up new avenues for research and development in this field.
Gielgud-O3
Understanding the diffusion mechanisms in solid electrolytes is crucial for the advancement of solid-state batteries. Our study employs ab initio molecular dynamics to investigate structural disorder in Li7–xPS6–xBrx argyrodites and its impact on lithium-ion conductivity. We challenge the traditional reliance on parameters like configurational entropy, bromide site occupancy, and bromine content as predictors of Li-ion diffusivity.
Our analysis reveals that high conductivity is primarily achieved through an equal distribution of bromine and sulfur across the 4a and 4d sublattices, which optimizes jump activation energies, lithium-anion distances, and charge distribution for enhanced ionic transport.
We introduce "ionic potential" as a novel, overarching descriptor that quantifies cation-anion interaction strength, effectively predicting argyrodite conductivity and aligning with experimental observations across various compositions. We demonstrate that minimizing and balancing ionic potentials at both sublattices (4a and 4d) greatly enhances conductivity by reducing anion-Li-ion interaction forces.
Additionally, this research deconvolutes the impact of sulfur/halide local environments on jump activation energy, explaining the role of site disorder in activating low-energy ionic pathways and providing crucial insights for designing high-performance solid electrolytes.
Fleming-K1
Montse Casas-Cabanas is the scientific coordinator of the Electrochemical Energy Storage Area and group leader of the Advanced Electrode Materials group at CIC energiGUNE. Her research interests focus on the design of battery materials and the understanding of phenomena that occur in energy storage devices through a multidisciplinary approach, with a focus in crystal chemistry.
She is also author of >75 scientific publications in peer reviewed journals and has been PI of several national and european projects. She has co-authored the FAULTS software for the refinement of X-ray data of crystalline structures with planar defects. She is also actively involved in the MESC+ Erasmus Mundus master course and has recently received the 2021 Young Researcher award ("Group Leader" category) from the Spanish Royal Society of Chemistry.
LiM0.5Mn1.5O4 (where M = Ni, Fe) compositions have emerged as a focal point of extensive research in the context of positive electrode materials for lithium-ion batteries. These materials, crystallizing in the spinel structure, have captivated significant attention owing to their remarkable ability to substantially enhance the average voltage compared to layered oxides or Mn-rich compositions, thereby holding great promise for advancing the performance of Li-ion battery systems. Moreover, these compositions are of particular interest due to their sustainable nature, being cobalt-free. This attribute aligns with the growing emphasis on eco-friendly battery technologies, contributing to a more sustainable and environmentally conscious approach to energy storage.
Exploring the intricate details of the crystal structures and compositional variations within this family of compounds becomes imperative for unlocking their full potential and contributing to the ongoing advancements in energy storage technologies. This study delves into a comprehensive analysis of the crystal chemistry of LiM0.5Mn1.5O4 (M = Ni, Fe) compositions, shedding light on their structural and microstructural details (including disorder and defects) and the pivotal role these play in enhancing the capacity and rate performance of these materials. [1-3]
Fleming-I1
One of the biggest challenges facing lithium-ion batteries is how to increase their energy density. The cathode, typically a layered lithium transition metal oxide, represents a major limitation. One route to increase the energy density is to store charge at high voltage on the oxide ions in the cathode material. However, removing electrons from lattice oxide ions typically results in structural instability leading to voltage hysteresis and voltage fade over cycling. Understanding the mechanism behind oxygen redox is critical to overcoming these issues.
Our recent investigations into Li-rich cathodes have revealed that oxidized oxygen takes the form of O2 molecules which are trapped in nanovoids in the structure. We have also shown that these trapped O2 molecules can be reduced back to O2- on discharge providing a viable charge storage mechanism to explain oxygen redox. In this talk, I will discuss the evidence for the formation and reduction of trapped O2 [1-3] and explore the impacts this has on the performance of oxygen redox cathodes[4]. I will show how the formation of O2 extends to 4d and 5d transition metal oxides[5], disordered rocksalt cathodes[6] and even to non-Li-rich cathodes[7]. Finally, I will show that it is possible to suppress this structural change and undergo reversible, high voltage O-redox without voltage hysteresis[8]. Altogether, this understanding helps to explain the unusual properties of oxygen redox cathodes and informs how they might be harnessed to boost the energy density of batteries.
Fleming-O1
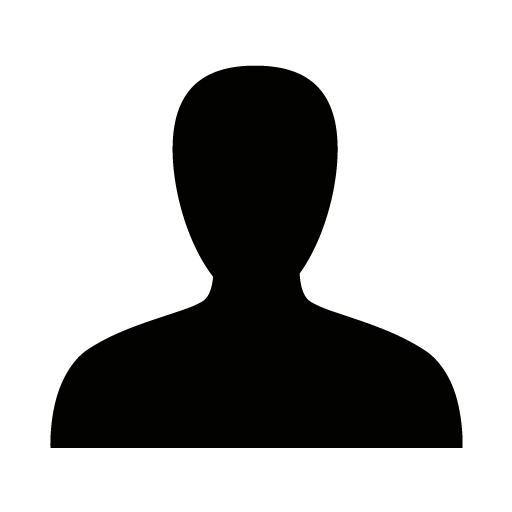
LiNi0.5Mn1.5O4 (LNMO) stands out as a highly promising spinel-type positive electrode material for Li-ion batteries (LIBs). Its high operating voltage (4.8 V vs Li+/Li, associated with the Ni4+/Ni2+ redox couple) provides high energy density, a crucial factor for the development of next-generation LIBs [1].
LNMO crystallizes with different extents of Ni/Mn ordering. In the fully ordered phase (P4332 space group), Ni and Mn cations occupy distinct crystallographic octahedral sites, whereas, in the fully disordered phase (Fd3m space group), Ni and Mn share the same crystallographic octahedral site. High temperatures (T > 750°C), required to obtain the disordered phase, lead at T ≥ 700°C to oxygen loss in LNMO and, as a result, to the formation of rock salt-based impurity and the reduction of Mn(IV) cations to Mn(III) [1,2].
The extent of Ni/Mn ordering significantly influences the performance of this positive electrode material. It is shown that the disordered LNMO exhibits superior performance at high C-rates and better cycling stability than the ordered LNMO [3,4]. Moreover, the disordered Mn-rich LNMO (LiNi0.44Mn1.56O4, Ni/Mn = 22/78) can be prepared without inducing oxygen deficiency, resulting in superior electrochemical performance [5]. This unexpected result encourages this systematic investigation of the impact of the Ni/Mn ratio on the phase equilibrium and electrochemical performance of LNMO. In this study, we performed ex situ and in situ Neutron powder diffraction under air and oxygen atmospheres to follow the Ni/Mn ordering process during the synthesis of LNMO with varying initial Ni/Mn of 25/75 (corresponding to stoichiometric LNMO), and Ni/Mn of 23/77 (Mn-rich LNMO).
Our findings reveal that for both Ni/Mn ratios, the Ni/Mn ordering process is disrupted by the oxygen release. In other words, the fully disordered LNMO phase cannot be prepared without rock salt-based impurities, caused by the oxygen release, for the selected Ni/Mn ratios. Our data also suggest that the extent of Ni/Mn ordering in LNMO is controlled solely by a concentration of Mn(III) in the crystal structure of LNMO, which can be adjusted by both the Ni/Mn ratio and partial oxygen pressure during the synthesis of LNMO.
Fleming-O2
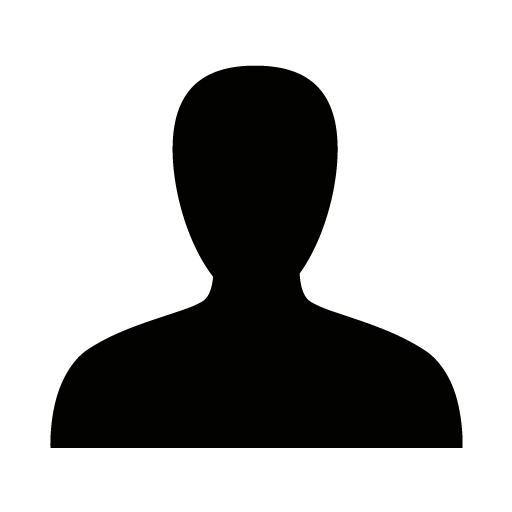
The development of high-energy-density batteries with lithium (de-)intercalation reaction is one of the most promising solutions to realize electric vehicles and power storage system applications with long-term stability. In this study, anti-fluorite structured Li5+xFe1-xMnxO4 was synthesized by a solid-state reaction, and lithium intercalation properties were characterized by electrochemical and structural investigations.
A mixed phase of the Li5FeO4-type and Li6MnO4-type structure was obtained for x < 0.6 composition. A new phase with the Li6MnO4-type structure was formed at x = 0.4, and a single phase with Li6MnO4-type structure was obtained in the range between 0.6 and 1.0 composition. The particle size of the synthesized samples was about 50 µm, which is quite large compared with conventional cathode materials for lithium batteries. The as-prepared Li5.6Fe0.4Mn0.6O4 exhibited first charge and discharge capacities of 680 and 300 mAh/g, respectively, with a large irreversible capacity of 380 mAh/g (coulombic efficiency = 44%). This indicates that the new iron-manganese composition with antifluorite-type structure is lithium insertion/extraction-active although a large irreversible electrode reaction was observed.
To improve the charge-discharge performance of the prepared sample, the electrode fabrication process was changed as shown in the experimental section. The ball milling process was introduced to decrease the particle size and compensate for homogeneous electronic conductivity in the composite electrode. Charge-discharge measurements showed that the first discharge capacity of the Li5.6Fe0.4Mn0.6O4 (x = 0.6) electrode treated by ball-milling was 450 mAh/g. The coulombic efficiency was 60%. A reversible reaction continued to proceed with ca. 200 mAh/g after the following cycles. A new iron-manganese system with an anti-fluorite structure could become a potential for high-capacity cathode material.
Westminster-K1
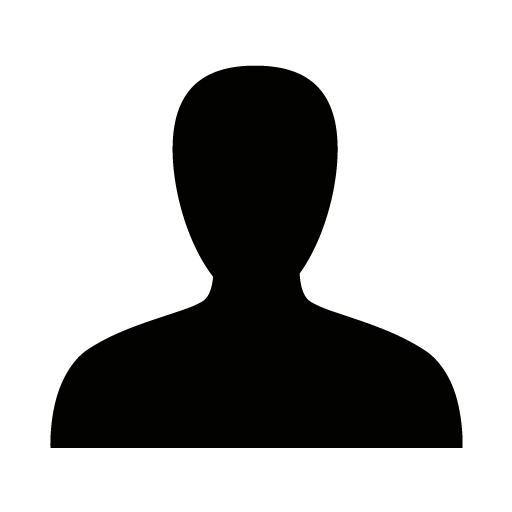
Li-ion batteries are ubiquitous; powering our cell phones, tablets, and laptop computers. Yet, fundamentally, we’ve exclusively relied on materials that adopt the same ordered rock salt structure type for over 20 years. During the time, we have continually optimized the performance of these materials to the point that we are rapidly approaching the theoretical limits on energy density. So where do we go from here? This talk will present an overview of the work our group has done to develop a deeper understanding of the fundamental structural and compositional requirements to effectively move charge through the solid state and discuss nascent work to develop reversible F-ion insertion hosts that function at room temperature. We will begin with an overview of the new design principles that must be applied in designing F-ion batteries and go on to highlight two major structural families that have been developed. We will conclude with a discussion of some of the challenges surrounding electrolyte development.
Westminster-I1
Introducing structural or compositional disorder within solid electrolytes is a common practical strategy for increasing their ionic conductivity. Dual cation (M, Sn)F2 fluorites have been proposed to exhibit two forms of disorder within their cationic host frameworks: occupational disorder between cations and orientational disorder of Sn(II) stereoactive lone pairs. To understand these two forms of disorder and their effect on fluoride-ion conductivity, we have performed a joint experimental and computational study of the exemplar cubic-BaSnF4, using X-ray diffraction, 119Sn Mössbauer spectroscopy, 19F NMR spectroscopy, and ab initio molecular dynamics simulations. We find that cubic-BaSnF4 shows extreme intrinsic fluoride-ion disorder, where 1/3 of fluoride ions occupy interstitial sites, with this disorder a consequence of repulsion between Sn lone pairs and fluoride ions. We also identify highly inhomogeneous fluoride-ion dynamics, where fluoride ions in Sn-rich environments are significantly more mobile than those in Ba-rich environments. Our simulations reveal that the Sn lone pairs dynamically reorient on a ps timescale, and that this lone-pair dynamics is coupled to the dynamics of local fluoride ions. These results illustrate how different forms of host-framework disorder can interact in an exemplar solid electrolyte to give complex mobile-ion dynamics, and highlight the important role of Sn(II) lone pair dynamics in this solid electrolyte.
Westminster-O1
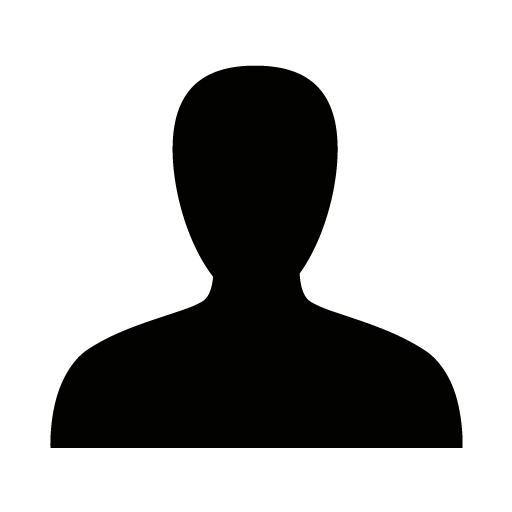
The vast majority of rechargeable batteries currently used for mobile electronics and vehicle applications are lithium-ion, exploiting their impressive energy densities and relatively high voltage. However, concerns around factors including safety, high cost and geopolitical distribution of the raw materials has motivated studies of other battery chemistries. Na+-ion cells are at the most advanced stage of development, driven by the high earth abundance of sodium, whilst multivalent mobile cations such as Mg2+ and Al3+ potentially offer increased energy densities, but are hampered by large structural changes associated with the insertion/removal of these highly charged species into/from the electrodes.
Until recently, batteries based on mobile anions (rather than cations) have been largely ignored, despite reports of high mobility of F- ions within solids dating back to Michael Faraday’s studies of the ‘fluoride of lead’, β-PbF2, in 1838. A major challenge for cells based on fluorine chemistry is the lack of a suitable liquid electrolyte, as most candidates contain HF2- ions with a small voltage stability window and/or react with atmospheric moisture to form HF. However, a significant advance occurred in 2011, with reports of viable cells using solid electrolytes such as BaSnF4 [1], which has recently attracted the interest of car companies including Honda and Toyota. However, these batteries have a major drawback, needing to be operated at elevated temperatures (typically 80-150oC) to achieve sufficiently high F--ion conductivity within the solid electrolyte [2].
This presentation will focus on the structure-property relationships within a number of F--ion conductors, including a discussion of our current understanding of the archetypal compound β-PbF2. This will be followed by a more detailed presentation of a number of ternary derivatives of PbF2, such as KPbF3 [3], PbSnF4 [4], CsSn2F5 [5] and β-KSbF4 [6]. Information on the crystal structure, including the nature of the dynamic F- disorder, has been provided by neutron powder diffraction studies, exploiting the sensitivity of the technique to the locations of the anions in the presence of heavier cations. Finally, the factors that promote extensive F- disorder within such solid phases will be considered, including the influence of crystal structure on the preferred anion diffusion mechanisms and the role of electron lone-pairs associated with cations such as Pb2+ and Sn2+ in promoting high F- mobility.
Westminster-O2
All solid-state fluoride ion batteries are promising and suitable for electric vehicles and grid storage due to their high energy density. The use of very high fluoride ion conducting solid electrolyte is necessary to achieve the potential of fluoride ion batteries. Various fluoride ion conducting solid electrolytes in the tysonite, and fluorite structured systems are under development. Among these fluoride ion conducting solid electrolytes the fluorite structured materials especially PbSnF4 system shows high ionic conductivity at ambient temperatures (~10-3 Scm-1). Similar to PbSnF4, the barium variant (BaSnF4) which exists in the same structure as PbSnF4, also shows conductivity in the order of 10-4 Scm-1 at room temperatures. In recent years, further increase in conductivity has been achieved by varying the barium to tin ratio. Especially, the solid solutions Ba1-xSnxF4 prepared by mechanical milling technique (x=0.5-0.57) with Sn rich ratio shows ionic conductivity nearly in order of 10-2 Scm-1 at room temperature. Therefore, we have studied the fluoride ion diffusion coefficient in this system since the diffusion coefficient plays a vital role in the mass transfer during battery operation. In the present work, the 19F diffusion coefficient in Ba1-xSnxF4 solid solution with high ionic conductivity has been studied using 19F pulsed field gradient nuclear magnetic resonance Spectroscopy (PFG-NMR). The fluoride ion diffusion coefficient is found to be in the order of 10-12 m2s-1 at room temperature. Further, we have investigated the fluoride ion conduction mechanism in Ba1-xSnxF4 by comparing the diffusion coefficient measured by 19F PFG-NMR and calculated from ac impedance measurements and the results will be discussed in detail.
Westminster-O3
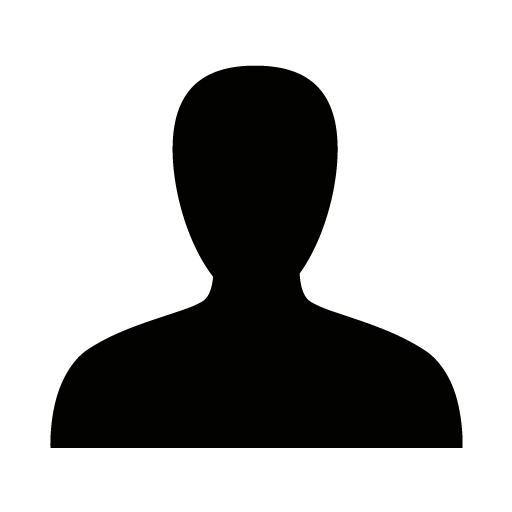
The development of high-performance fluoride-ion (F−) conductors is essential for the practical application of all-solid-state F− batteries. To date, the crystal structures of the F− conductors have been mainly limited to perovskite-, fluorite- and tyssonite-type fluorides. However, the room temperature conductivity of these F− conductors is several orders of magnitude lower than that of cationic conductors. Furthermore, strategies to improve F− conductivity are mainly limited to introducing defects in the crystal structure, which lags considerably behind the abundant means of conductivity enhancement in cationic conductors, such as inductive effects1 and paddlewheel effects2.
In mixed-anion compounds that containing more than one anion in the structure, the presence of anions with different polarization rates and electronegativity from F− allows the construction of new crystal structures that cannot be obtained in single-anion compounds3. In this study, F− conduction in fluorosulfide La2SrF4S2 was investigated[4]. In La2SrF4S2, the triple-fluorite layer La2SrF4 allows for a two-dimensional F− conduction, which is not found in single anion fluorides. The presence of a sulphur layer widens the conduction space of the F− layer and weakens the interaction between cations and F−. Furthermore, we attempted to improve the F− conductivity by cation-anion engineering. Specifically, (i) La2SrF4+xS2-xClx, in which S2− was changed to Cl− to introduce excess F−, and (ii) La2Sr1-xPbxF4S2, in which Sr2+ was changed to Pb2+ with 6s2 lone pair electrons, were synthesized and the change in F− conductivity were explored and explained from a crystallographic point of view.
(i) The ionic conductivity of La2SrF4.1S1.9Cl0.1 at 160 °C was 6.26 × 10−6 S cm−1, which is five times higher than that of La2SrF4S2. On the other hand, the activation energy of La2SrF4.1S1.9Cl0.1 is 0.51 eV, which is comparable to 0.53 eV of La2SrF4S2. The increase in the pre-exponential factor can be attributed to the introduction of excess F− in the lattice by replacing divalent S2− with monovalent Cl−. This suggests that the use of mixed-anion compounds allows carrier engineering by anions, which is different from the carrier control of by cation substitution in previous F− conductors.
(ii) The ionic conductivity of La2Sr0.6Pb0.4F4S2 at 160 °C was 2.56 × 10−4 S cm−1, which are both higher than those of La2SrF4S2 and La2SrF4.1S1.9Cl0.1. The amount of F− carriers and the F−-F− distance did not change much before and after the introduction of Pb2+, suggesting that there are factors other than those previously considered responsible for the increased pre-exponential factor. 19F MAS NMR showed that the number of F− in the hetero-cationic environment increased after the introduction of Pb2+, suggesting that the distorted cationic sublattice is partly responsible for the conductivity enhancement. Also, 6s2 lone electron pairs in Pb2+ may also contribute to the increase in F− conductivity.
In conclusion, using the mixed-anion compound La2SrF4S2 as a model, we proposed new strategies to improve F− conductivity by cation-anion engineering.
St.James-K1
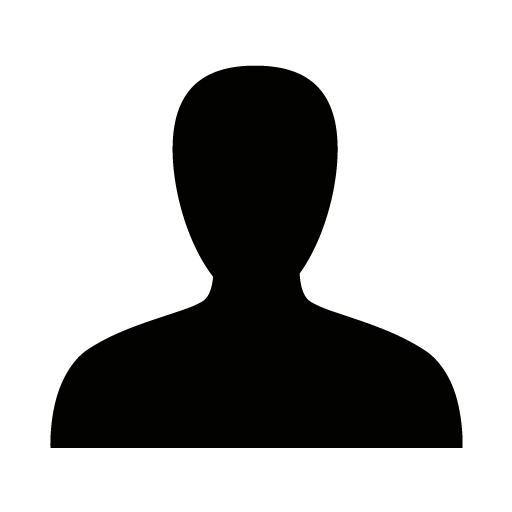
One of the major problems with proton-conducting oxide fuel cells is the large chemical expansion upon hydration and the resulting mechanical stress within the electrolyte. The purpose of this study was to establish a method for numerical calculation of stress distribution under fuel cell (and electrolysis) operating conditions and a method for experimental verification of the estimated results. In this study, Ba(Zr,Y)O3 and Ba(Zr,Yb)O3 were selected as the target proton-conducting oxides. First, the lattice volume change was modeled using published thermodynamic parameters of defect equilibrium, along with experimental results from high-temperature X-ray diffraction. Although a significant change in lattice volume was observed upon hydration, the influence of redox reactions was found to be negligible. Then the elastic modulus of the material was measured using a resonance method at elevated temperatures in controlled atmospheres. The chemical potential distribution of oxygen and hydrogen (and water vapor) under fuel cell (or electrolysis) conditions were estimated using an equivalent circuit containing three transmission lines, for proton, oxide ion, and hole, terminated by gas-solid exchange reaction resistors for oxygen, hydrogen, and water. The obtained proton concentration distribution was used to calculate the chemical strain distribution across the sample. The stress distribution and deformation of the electrolyte were calculated for the free-standing and constrained states. The information of the deformation of the free-standing sample was used for experimental validation of the calculated results. In the verification experiment, a sample pellet with Pt and Pd electrodes on the air and fuel sides of the surface was placed on a quartz tube with using mica as a gas sealant, and the surface profile was measured using a reflection-type laser profilometer. When the water vapor partial pressure on the air side was increased, the sample deformed concavely toward the fuel side. This change was confirmed reversible by increasing and decreasing the water vapor pressure. The amount of deformation agreed well with the calculated value, confirming that the calculated results were valid.
St.James-O1
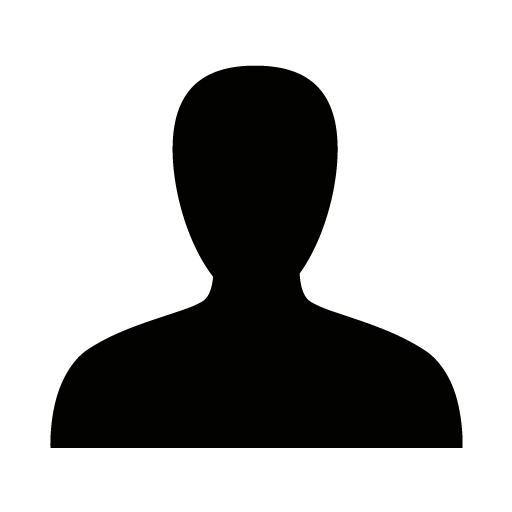
This research focuses on the interplay between the processing conditions, structure, transport, and proton surface exchange kinetics, of vertically aligned nanocomposites (VANs), targeting protonic ceramic electrochemical cells (PCECs). The VANs consist of a proton conductor (BaZr0.9Y0.1O3-δ, BZY) as the first phase and a mixed p-type/ oxide-ion conductor (Ce0.9Pr0.1O2-δ, PCO) as the second phase. By separating the proton-conducting and p-type mixed conducting phases for task sharing, the BZY-PCO VANs possess the potential for rapid proton surface exchange at the solid-gas interface and transport along the solid-solid heterointerfaces.
These VAN thin films were deposited on MgO (100) substrates with pulsed laser deposition (PLD) under different conditions. Owing to the small lattice mismatch between MgO and BZY, along with the different crystal structures of perovskite BZY and fluorite PCO, it is expected that this combination can lead to vertically aligned phase-separated structures. To optimize the PLD recipe for high-quality VANs, we varied the substrate temperature, laser repetition rate, laser power, and processing oxygen pressure. The crystallinity and phases were characterized by grazing-incidence X-ray diffraction (GI-XRD), and the structural analysis and elemental mapping were performed by scanning/transmission electron microscopy (S/TEM), energy-dispersive X-ray spectroscopy (EDS), and electron energy-loss spectroscopy (EELS). Additional characterization of strain and structural order as a function of depth was enabled by angle-dependent synchrotron X-ray pair distribution function (PDF) analysis.
Furthermore, proton surface exchange kinetics of VANs were evaluated by simultaneous electrical conductivity relaxation (ECR) and optical transmission relaxation (OTR) measurements. This research reveals how processing conditions influence mesostructural ordering in VANs, heterointerface chemistry, and the kinetics of proton surface exchange.
St.James-O2
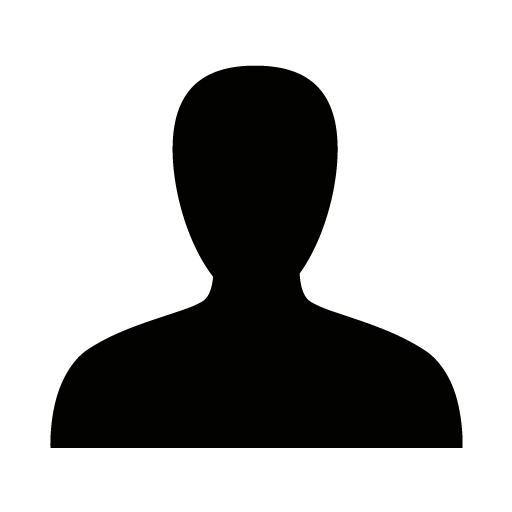
Acceptor-doped barium zirconates are of major interest as proton conducting electrolyte materials for electrochemical applications at intermediate operating temperatures. The conduction of protons through polycrystalline yttrium doped barium cerium zirconates (BZCY) is hindered by the formation of space charge regions at grain boundaries caused by a positive core charge. During high temperature sintering, the positive core charge additionally acts as a driving force for acceptor dopant segregation. Yttrium segregation to grain boundaries has been observed in sintered ceramics but the fundamental relationships between the degree of segregation and protonic conductivity are not explored. Here, we present a comprehensive study of the influence of yttrium segregation on the chemical composition and structure at grain boundaries in BZCY and its impact on electrochemical properties. We designed an out-of-equilibrium model material, that displays no observable segregation and used it as a starting point to observe the kinetics of segregation and the induced changes in grain boundary conductivity after varied thermal histories. Furthermore, we coupled the electrochemical results derived from impedance spectroscopy to atomic resolution transmission electron microscopy. We discovered that atomically sharp acceptor segregation drastically increases the grain boundary conductivity both in the model system and reference samples processed by the industrially applied solid state reactive sintering (SSRS) route.
St.James-O3
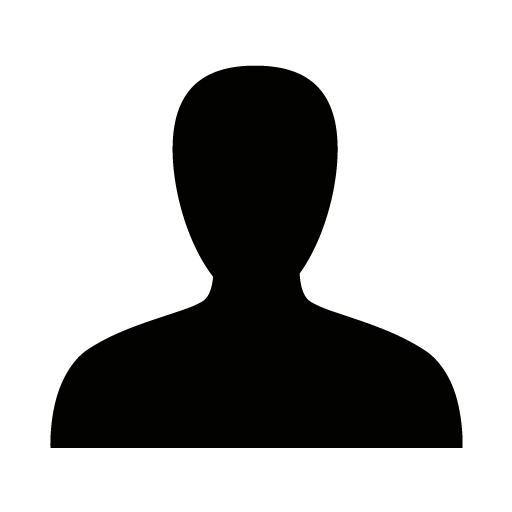
For decades, much effort has been devoted to developing proton conductors applicable to electrochemical devices at intermediate temperatures. However, promising materials that possess sufficient conductivity have not been realized yet. This study demonstrates the development of novel proton conductors that are operative at intermediate temperatures, especially 300–400 °C, through the simple ion-exchange method. The Li+/H+ ion-exchange process was conducted for Li(14−2x)/4Zn(1+x)/4GeO4 (lithium super ionic conductors, LISICONs) in non-aqueous solutions. The chemical formula of the resultant sample was determined as Li3.13H0.37Zn0.25GeO4. The electrical conductivity of this material was evaluated to be 87.0 mS cm−1, 39.0 mS cm−1, and 5.5 mS cm−1 at 400 °C, 300 °C, and 200 °C, respectively, in 10% H2O–90% N2. Furthermore, the main charge carrier in this electrolyte was identified as a proton from the H/D isotopic exchange study. These findings open up the possibility of realizing new electrochemical devices that are operative at 200–400 °C.
Moore-I1
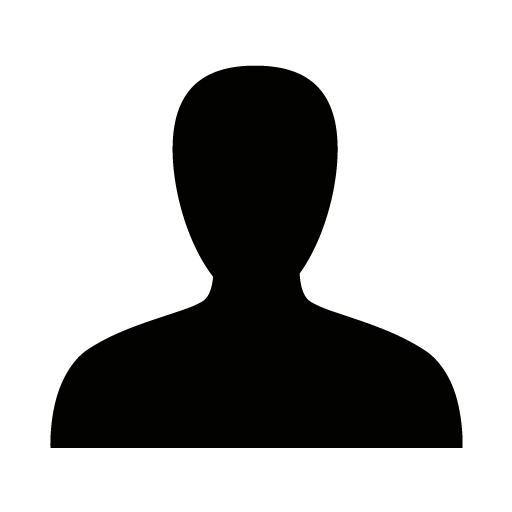
Solid-state batteries have the potential to be a disruptive technology because of their ability to improve safety and increase energy density by incorporating Li metal anodes. However, all solid-state interfaces present unique challenges, including high interfacial impedances, accommodation of mechanical stresses due to solid-solid interfacial contact, and (electro)chemical instabilities that can evolve during dynamic cycling conditions. Furthermore, a significant challenge facing the scale-up of SSBs is to improve our understanding of processing science needed to enable manufacturing.
To address these challenges, our group focuses on gaining new fundamental insights into the coupled phenomena occurring at interfaces, and applied this knowledge to rationally design interfacial composition and structure to address the root cause of performance limitations. As an enabling technology, we have developed operando video microscopy methods that allow for a dynamic visualization of the morphological and electrochemical evolution of solid-state electrochemical interfaces.
In this talk, I will describe our journey to deepen our understanding of interfacial phenomena at both the Li metal anode side, as well as in composite solid-state cathode materials. On the anode side, there is a critical need to understand and control the coupling between electrochemistry, morphology, and mechanics, to improve the uniformity and reversibility of plating and stripping during charge and discharge, respectively [1-4]. On the cathode side, we focus on overcoming energy/power tradeoffs, which exhibit unique phenomena owing to the single-ion conducting nature of ceramic electrolytes, as well as improving stability at high voltages [5]. Throughout the discussion, a common theme will be the identification of electrochemical signatures of the coupled thermodynamic, kinetic, and transport phenomena that occur at solid-state battery interfaces. Finally, equipped with this fundamental knowledge, I will discuss strategies to rationally design and manufacture optimized electrode architectures to improve stability and reversibility during cycling.
Moore-O1
Dr. Jongwoo Lim currently serves as an Associate Professor of Chemistry at Seoul National University. His academic journey began at POSTECH, where he obtained his undergraduate degree in Chemistry in 2008. He later pursued his Ph.D. in Chemistry from the University of California, Berkeley, graduating in 2013 under the guidance of Professor Peidong Yang. Post-Ph.D., Dr. Lim engaged in postdoctoral research with Professor William Chueh at Stanford University, before joining the Department of Chemistry at Seoul National University in 2017 as an Assistant Professor.
In the conventional view, electrode kinetic properties are obtained ex-situ near equilibrium, where the lithium concentration is only slightly perturbed. However, during high cycling rates, lithium transport within the electrode creates substantial lithium concentration differences, leading to dynamic phase separation. Therefore, we discovered that conventional kinetic properties fail to assess the effective rate capability at fast C-rates. Instead, kinetic phase heterogeneity, characterized by dynamic separation observed in-situ with X-ray diffraction (XRD) and deviations in lattice parameters, exclusively correlates with the capacity at high C-rate cycling.
To understand such phase evolution or separation, it is crucial to reveal the lithium transport pathway within the individual crystalline particles or grains. Using operando X-ray microscopy technology, our group has unveiled how lithium inserts and de-inserts within individual crystalline battery particles during cycling. We note that lithium transport and phase evolution behavior on the surface are critically different from those in the bulk and also play a crucial role in determining the rate capability.
By performing electrode-level operando XRD and single-particle-level operando X-ray microscopy, lithium insertion and de-insertion kinetics could be elucidated much further, aiding the understanding of fast battery cycling protocols.
Moore-O2
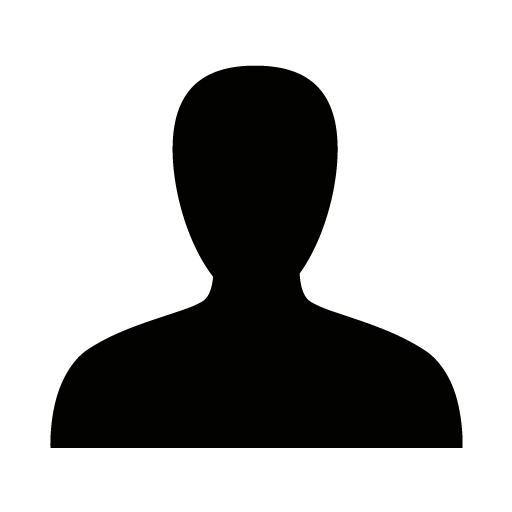
Interfaces have been a key region of interest in solid-state batteries for many years. The dynamic interplay between the solid-electrolyte and the respective anode defines crucial characteristics of the resulting Solid-Electrolyte Interphase (SEI), particularly in terms of chemical states and acts as a key indicator of the solid-electrolyte’s suitability for a specific battery system. Understanding the fundamentals of the SEI formation is paramount in developing next-generation batteries. Previous studies of interface characterisation have been confined to ex-situ analyses, which require numerous repetitions to yield reproducible and representative data for comparison with industrial systems. In this work, we present an in situ characterisation method for interfaces, that is simple and adaptable to various sample conditions such as, as-prepared material or electrochemically tested samples.
In this work, the solid-electrolyte used was based on the NASICON composition with a stochiometry of Na3.4Zr2Si2.4P0.6O12 synthesised through solution-assisted solid state reaction. In situ metallic anode|NASICON interface was formed by inducing negative charge across the analysis area of the sample, to form a ‘negative’ electrode, through the applied combination of flood gun and primary-ion gun within a Time-of-Flight Secondary Ion Mass Spectrometer (ToF-SIMS). This created charge imbalance between the ‘negative’ electrode and metallic anode, promoting the diffusivity of Na+ ions. To facilitate the mobility of charge carriers, and provide optimal connection to both negative and positive electrode, samples were setup in an in-house metallic casing. Ion maps and profiles were obtained using the same ToF-SIMS instrument, and cross-validated to results obtained via ex situ X-Ray Photoelectron Spectroscopy.
Evolution in the chemical states of the sodium anode|NASICON interface was observed indicating an interphase formation, suggested to be Na2ZrO3. The condition of the sample, as-prepared or electrochemically tested, was also found to influence the homogeneity of the Na+ plating, thus resulting in direct observation of metal filament/dendrite propagation. Our findings demonstrate that Na+ mobility can be controlled to induce galvanostatic charge/discharge cycling, that effectively mimick a battery’s mode of operation and the SEI formation that would occur in a conventional symmetric cell. This work not only advances our understanding of solid-electrolyte interfaces using NASICON in sodium-ion batteries but also presents a practical and versatile approach to in situ characterisation, laying the foundation for the development of more efficient solid-electrolytes for next-generation batteries.
Moore-O3
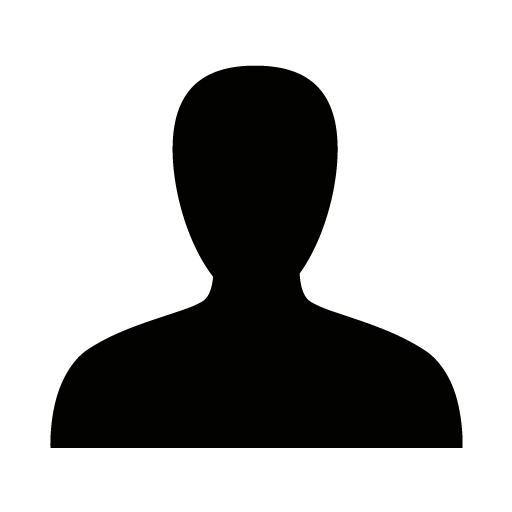
All-solid-state batteries (ASSBs) are a rising alternative for boosting the volumetric energy density and are considered safer than conventional Li-ion batteries. However, Li-ion transport across the solid electrolyte (SE)/active materials (AMs) interfaces is limited by the parasitic (electro-)chemical side reactions responsible for the impedance rise and lower performance. The fundamental understanding of such interfaces has not been fully achieved yet, mainly due to the limitations in current surface-sensitive analytical techniques, especially in operando mode.
In this contribution, we will report the recent developments in operando X-ray photoelectron spectroscopy (XPS)[1], X-ray absorption spectroscopy (XAS)[2], and X-ray photoemission electron microscopy (XPEEM)[3] to monitor in real-time the interface evolution of operational ASSB working electrodes (WE) at multiple measurement length scales. Operando measurements are made possible thanks to the unique and versatile electrochemical custom-made cells designed to operate in ultra-high vacuum, providing reliable electrochemistry.[4] We will highlight how the combination of in-house operando XPS and synchrotron-based operando XAS/XPEEM using soft and tender X-rays can provide a reliable platform to investigate (i) the (electro‑)chemical evolution of the SE/AMs interfaces, (ii) the surface modifications of the AMs, and (iii) the electronic properties across electrified solid-solid interfaces. Different examples will be presented describing in a novel way the interface degradation mechanism observed in real time upon cycling.
Specifically, we will show results from operando XPS performed on WE made of ball milled Li3PS4 (LPS) mixed with VGCF conductive carbon. We identified accurately, by combining cyclic voltammetry and operando XPS, the onset potentials of the LPS oxidation and reduction, and the various formed byproducts species, when cycled in high voltage [2.4 V - 5 V vs. Li+/Li] and low voltage [2.4 V – 5 mV] ranges. We demonstrate the non-reversibility of the polysulfide with bridging sulfur species formed in the high voltage range during the 1st charge, and the reversibility of the Li2S and LixP species formed in the low voltage range over multiple cycles.
In operando XAS, we performed measurements at LiNi1/3Co1/3Mn1/3O2 (NCM111) and LPS WE by acquiring the Mn, Ni, and Co L-edge spectra, as well as at the S and P K-edge spectra in both total electron yield (TEY) and total fluorescence yield (TFY) modes.[5] From the TEY surface sensitive mode (~10 of nm), we detected the formation of electrochemically inactive NCM surface rich in reduced Ni2+, Co2+, and Mn3+ transition metals co-responsible for the impedance rise, together with Li3PS4 oxidized byproducts. Additionally, from the TFY bulk sensitive mode (100s of nm) the charge compensation mechanism and the onset potentials during the NCM111 delithiation is elucidated by following the Ni and Co L-edges oxidation evolution to +4 state up to 4.5 V vs. Li+/Li. Interestingly, by monitoring the O K-edge we evidence the oxygen oxidation above 4.5 V and its reversible involvement in the charge compensation process. [6]
Finally, with the support of operando XPEEM performed on both NCM622 and LiNbO3 coated NCM622 mixed with Li6PS5Cl (LPSCl) as WEs, we elucidate the missing piece of the exact electrolyte-electrode degradation mechanism and the role played by the LiNbO3 coating to reduce the impedance. Thanks to the excellent nanoscale lateral resolution better than 70 nm and the local spectroscopic capability on single NCM particles, with a depth of analysis of 3-4 nm, we revealed that the reduced transition metals formed at the cathode surface are mainly initiated by the layered oxygen loss which is further enhanced in contact with the LPSCl. Interestingly, we confirm that the presence of LiNbO3 as coating mitigates the formation of the reduced transition metal and improve the Li kinetics at the interface with the SE.
Abbey-K1
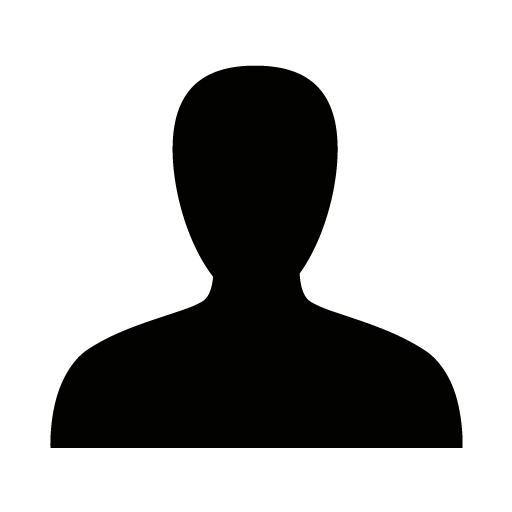
The surface segregation of cations, particularly strontium (Sr), has been identified as a key factor contributing to the performance degradation of perovskite-based oxide electrodes used in various energy conversion devices. However, the complex chemistry and structure of the perovskite oxide surfaces have led to only partial comprehension of the mechanisms behind Sr segregation and its impact on electrode activity. Moreover, Sr segregation already occurs during perovskite synthesis, further complicating the situation.
To address this issue, this study implements a controlled approach using a model thin film system composed of atomically flat SrTi0.5Fe0.5O3-δ (STF50) with a stoichiometric surface, enabling detailed examination. The evolution of surface structure, composition, and oxygen exchange kinetics are observed as a function of temperature and time. By integrating experiments and ab initio simulations, we tackle several fundamental questions, including evaluating reactivity for pristine perovskite oxide surface before Sr segregation and the correlation between Sr segregation at the surface with oxygen exchange kinetics. Our comprehensive analysis reveals that the decline in performance of the perovskite oxide electrodes is primarily attributed to the detrimental effects of Sr deficiency on the surface, thereby resolving longstanding debates in the field.
Abbey-I1
In the last years exsolved nanoparticles from perovskite oxide matrices demonstrated to be high performance catalysts due to their thermal stability, sintering resistance and regenerability. Particular focus has been placed on the development of FeNi bimetallic exsolved systems due to their higher catalytic activity and product selectivity [1,2]. One of the reasons for their higher performance was attributed to the formation a defective crystalline FeOx shell around a FexNiy core [1]. Recently, we demonstrated that the exsolved Fe:Ni ratio can be controlled by the extent of A-site deficiency in the parental La0.4Sr0.6-xTi0.6Fe0.35Ni0.05O3±δ (0 ≤ x ≤ 0.2) matrix thus providing catalytic systems with tunable selectivity with respect to the CO2-mediated partial oxidation of ethane [2].
In the present report, we show that the exsolved Fe:Ni ratio and so the catalytic selectivity in La0.4Sr0.4Ti0.6Fe0.35Ni0.05O3±δ can also be controlled by the exsolution temperature and how these processes can be fully switched. Low reduction temperature (T = 400 °C) favors the formation of Ni-rich nanoparticles (NiFe), whereas at high temperature (T = 850 °C) the exsolution of Fe is predominant (FeNi). The combination of spectroscopic analyses (Mössbauer, XANES) with XRD and in situ HR-TEM provided full explanation of the temperature-dependent exsolution process. For the CO2-mediated partial oxidation of ethane, NiFe nanoparticles were selective for the dry reforming pathway by 95%, because of their high metallic character. On the contrary, the FeNi system showed very high selectivity (over 70%) for the oxidative dehydrogenation, i.e. ethylene formation, due to the formation of a defective crystalline wustite (FeO1+x) shell as demonstrated by TEM and Mössbauer spectroscopy analyses. Long-term catalysis tests revealed the high performance stability of bimetallic exsolved nanoparticle, due to the poor formation of carbon depositions.
Controlling the nanoparticle composition using a process parameter like temperature presented us a unique opportunity: to switch between the NiFe and FeNi nanoparticles in a single matrix through the oxidation step (Figure 1). We demonstrated that this is fully achievable and that both nanoparticle properties and their catalytic selectivity are interchangeable in a single perovskite compound. This opens up to a completely new application landscape for exsolved materials in catalysis and further show the manifold opportunities that the exsolution process offers for designing advanced energy materials.
Abbey-O1
Symmetrical Solid Oxide Cells (SOCs) are one of the most interesting energy conversion and storage devices. The concept of symmetrical construction is associated with the use of same compounds as the anode and cathode, which can decrease the number of cell components and simplify the fabrication and later recycling processes. However, electrode materials for symmetrical design must meet certain requirements, such as good structural stability in reducing and oxidizing atmospheres, and excellent electrocatalytic activity towards fuel oxidizing and oxygen reduction [1-4]. The application of materials with the ability to in situ exsolution of nanoparticles in the SOCs electrodes is considered to be highly beneficial, allowing for the improvement of catalytic activities and stability [4]. Moreover, nanofiber-structured electrodes with highly favorable microstructure including continuous porosity and more reaction sites, efficiently contribute to a significant enhancement of the electrochemical performance of SOCs, especially in lower work temperature ranges [5].
In this work, the A-site deficient (Sm/Nd)0.9Ba0.9Mn1.8-xFexNi0.1Co0.1O6-δ perovskites with double perovskite structure (A-site cations ordering, P4/nmm space group) were successfully synthesized by the soft chemistry method. The obtained double perovskites can potentially present excellent ionic diffusion properties due to the layered structure. A continuous phase transition from P4/nmm to P4/mmm was recorded for the studied materials at around 200 °C, characterized by the second order. The measured thermal expansion coefficient (TEC) values are very close to TECs of mostly used electrolytes (including La0.9Sr0.1Ga0.8Mg0.2O3−δ and Ce0.8Gd0.2O2−δ). The obtained (Sm/Nd)0.9Ba0.9Mn1.8-xFexNi0.1Co0.1O6-δ compounds show excellent redox stability, favoring their application as potential electrode materials for symmetrical SOCs. It has been found that the A-site nonstoichiometry (Sm0.9/Nd0.9Ba0.9) favors the in situ exsolution of metallic nanocatalysts from the parent materials in reducing conditions, therefore boosting the electrochemical performance of SOCs. The presence of in situ exsolved nanoparticles (CoNix and CoNixFey) was confirmed on the surface of reduced compounds by the SEM and HR-TEM studies. Interestingly, the dissolution of exsolved nanoparticles to the parent material was also observed in the oxidizing atmosphere at elevated temperatures. Nanofiber-structured electrodes fabricated by the electrospinning technique, significantly impact the exsolution process of nanoparticles, and boost the electrochemical properties of constructed SOCs. Excellent electrochemical performance of the proposed perovskite electrodes was recorded in the reducing condition. Sm/Nd0.9Ba0.9Mn1.8-xFexNi0.1Co0.1O6-δ-based symmetrical cell exhibits as low as 0.005 Ω·cm2 electrode polarization at 850°C in 5 vol. % H2/argon, which indicates that the investigated perovskite is particularly of interest for the application as anode material.
Abbey-O2
Alfonso J. Carrillo holds a Ph.D. in chemical engineering by Universidad Rey Juan Carlos (Spain)—research conducted at IMDEA Energy. Then, he moved to the Electrochemical Materials Laboratory, first at ETH Zurich (Switzerland), and after at MIT (USA), where he was 2018 Eni-MIT Energy Fellow. He has been awarded with the Energy and Environmental Research Grant by Fundación Iberdrola, Juan de la Cierva Formación by the Spanish Ministry of Science, and Junior Leader Fellowship by Fundación LaCaixa. He has worked at ITQ (Spain) since January 2019, with a focus on the functionalization of redox oxides for energy storage and production of renewable fuels.
The exsolution process from perovskite oxides has emerged as a promising alternative to achieve highly dispersed and stable metallic nanoparticles by annealing in a hydrogen-containing atmosphere. The great advantage is that exsolved metallic nanoparticles remain anchored to the oxide backbone, preventing sintering [1]. Besides, they improve electrode resistance against carbon deposition, providing a better long-term performance under catalytic tests compared to commercial noble metal-supported catalysts. Additionally, by careful compositional control, it is possible to exsolve metallic alloy nanoparticles in a facile manner, which can trigger unprecedented electrocatalytic properties.
This work focuses on the exsolution of multicomponent metallic Ni-Co-Fe alloys from Sr2FeCo0.2Ni0.2Mn0.1Mo0.5O6-δ perovskite electrodes in which we carried out a microstructural optimization by a careful adjustment of the exsolution treatment conditions. In a previous work, these materials were tested as fuel electrodes for CO2 electrolysis, exhibiting high Faradaic efficiencies and lower polarization resistance when functionalized with ternary alloy exsolved nanoparticles of 10 nm size [2]. Here, we prove that by carefully choosing the exsolution processing conditions (temperature and pressure), it is possible to further adjust the Ni/Co/Fe content of the exsolved nanoparticles. Interestingly, high-pressure exsolution (up to 100 bar), unexplored to date, was found to have a volcano-like trend, for a fixed temperature, on both the exsolution extent and the elemental composition of the nanoparticles, which helped in identifying the optimum pressure. Additionally, high pressure allowed for exsolution at low temperatures, resulting in high nanoparticle dispersions (ca. 7000 particles /μm2) at 300 ºC, which were one order of magnitude higher than at 600 º C.
In summary, this talk will provide useful processing guidelines for further development of multi-component metallic exsolution that could be extended to other perovskite compounds or other oxides (fluorites, spinels) to design more efficient exsolved nanocatalysts.
Abbey-O3
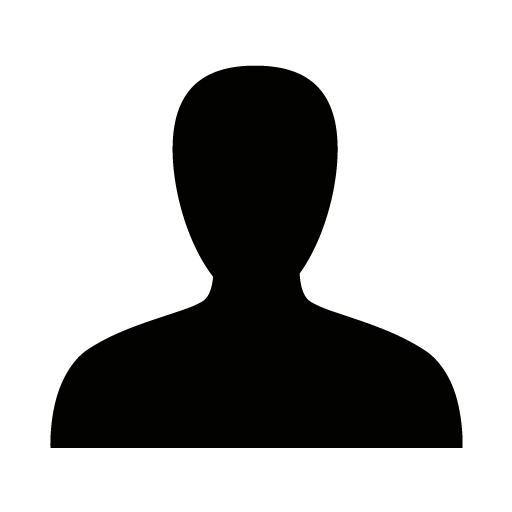
To address the ongoing environmental crisis, hydrogen emerges as an appealing pathway for facilitating large-scale decarbonization efforts. Concurrently, the development of highly efficient devices is imperative, and Solid Oxide Fuel Cells (SOFCs) present significant potential in this regard. Presently, hydrogen storage stands as a major bottleneck hindering the widespread adoption of this technology. Hence, ammonia emerges as a promising hydrogen carrier due to the presence of well-established plants, its carbon-free nature, and a narrower flammability range compared to hydrogen and hydrocarbons. Nonetheless, the realization of materials and devices tailored for operating SOFCs with ammonia fuel must be strategically planned.
The utilization of supported nanoparticles systems has garnered increased attention for their versatility and potential to achieve advanced performance across various applications including chemical conversion, and electrochemistry. The exsolution of single metal/alloy nanoparticles represents an emerging method to address common issues associated with nanomaterials, such as chemical poisoning or coalescence at high temperatures, owing to their robust interaction with the support material. Recent studies have revealed that the environmental conditions strongly influence the morphology of exsolved nanoparticles, thereby offering opportunities to tailor their activity for specific applications.
Hereby, we synthesized and studied titanates with different A-site deficiency and increasing Fe content in B-site in order to increment the exsolution and reducing the working temperature, respectively. Indeed, samples, (La0.50Sr0.50)1-αNi0.05Ti1-xFexO3 with α = 0.10 and 0.20 and x = 0.05, 0.35 and 0.60, convert ammonia in hydrogen and nitrogen without production of NOx in 400 – 750 °C range. Prepared samples were firstly characterized structurally and morphologically by X-Ray Diffraction (XRD) and Scanning Electron Microscopy (SEM) to study the dispersion of NPs on the surface and their chemical nature (Fe and NiFe NPs). Durability tests were performed in order to preliminary evaluate the corrosive effect of ammonia on the powders that detect the presence of superficial nitrogen though XPS measurements. Electrochemical tests were performed by preparing button cell prototype via screen printing using both hydrogen and ammonia as fuel. Impedance Spectroscopy was exploited to identify physic processes involved in ammonia decomposition respect to pure hydrogen in a wide temperature range (400-900°C) to also understand the role of A-site deficiency and Fe content on electrochemical properties. Finally, spent cell prototype were investigated by microscopies to study damages brough by ammonia.
Gielgud-K1
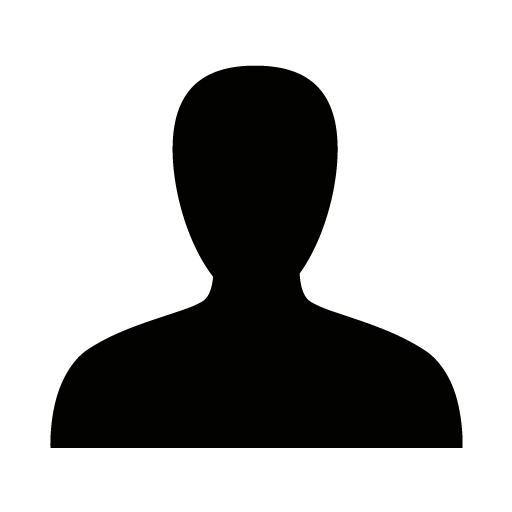
Electrostriction is a second order electromechanical response present in all solid dielectrics. According to the scaling law derived more than two decades ago by Prof. R. Newnham (Penn State), the hydrostatic electrostriction polarization coefficient (Qh) for a wide range of classical electrostrictors scales with the ratio of the elastic compliance to the dielectric permittivity.
Since 2012, a number of ion conducting ceramics and thin films have been reported to exhibit longitudinal electrostriction strain coefficients (M33) much larger than that predicted by the classical scaling law, i.e., non-classical electrostriction (NCES). These include such well-studied oxygen ion conductors as trivalent rare-earth doped ceria, (Nb,Y)-stabilized cubic Bi2O3 and proton conducting, acceptor-doped BaZr1-xXxO3-x/2+δH2δ ceramics, where X= Ga, Sc, In, Y or Eu, and 0.05 ≤x≤0.2. For these materials, the longitudinal electrostriction strain coefficient |M33| is >10-17 to 10-16 m2/V2, i.e., 20-1000 times larger than expected and has been shown to originate in elastic dipolar strain induced by point defects. According to EXAFS measurements and DFT modeling, polarizable elastic dipoles originate from local lattice distortion around oxygen vacancies involving both the first and second coordination shells. The resulting strain field has a characteristic interaction length ≤ two - three unit cells (1.4nm).
Under ambient conditions, |M33| for oxygen ion conductors decays by more than two orders of magnitude at frequencies above ~1 Hz, and, as has been found for intermediate temperature ionic conductivity, reaches a maximum for approx. 10 mol% Gd- and Sm- doped ceria. This correlation suggests that ionic conductivity and NCES electrostriction may have similar underlying physical origins, which impart mobility to oxygen vacancies in an electric field while allowing elastic dipoles to reorient. Unexpectedly, oxygen ion conductors contract parallel to the electric field with fully recoverable saturation strain < -15 ppm. However, application of DC bias ~7.5 kV/cm to 5-10 mol% Sm- or Gd- doped ceria pellets for > 14 hours produces positive strain at the ceramic surface layer of ~ 600 ppm; strain falls with higher dopant content, paralleling the decrease in |M33|. Following bias removal, strain persists at room temperature for at least 24 hrs. Upon heating to 140 °C for 5 hours, the unstrained state is recovered. This cycle of field-induced expansion and annealing can be repeated multiple times, providing evidence for some type of order-disorder transition of the vacancy-induced elastic dipoles. According to our current understanding, reorientation of elastic dipoles under an electric field may be related to either dielectric and/or elastic compliance anisotropy, as vacancy-induced elastic dipoles do not have a permanent electric dipole moment.
For anhydrous, acceptor-doped BaZr1-xXxO3-x/2+δH2δ, |M33|= (1-7) ·10-16m2/V2 below, and ≈10-18-10-17m2/V2 above, the Debye-type relaxation frequency. Hydration by ~60% does not significantly affect the magnitude of |M33|, but does increase the relaxation frequency by a factor of 10 to 100, suggesting that, in the presence of interstitial protons, NCES can respond more rapidly than those based solely on oxygen vacancies.
Such a combination of large longitudinal electrostriction strain coefficient, low dielectric permittivity and large elastic modulus, as observed in NCES, emerges due to elastic and dielectric properties which are largely defined by the host lattice, while electrostrictive strain is controlled by the strength and polarizability of the elastic dipoles. We suggest that NCES may be a common feature for polycrystalline, solid dielectrics containing mobile point defects. NCES may also emerge in materials with isovalent doping, e.g., Zr-doped ceria, if the difference in ionic radius of the host and dopant cations is sufficiently large that local instability results, leading to dynamic elastic dipole formation.
Gielgud-I1
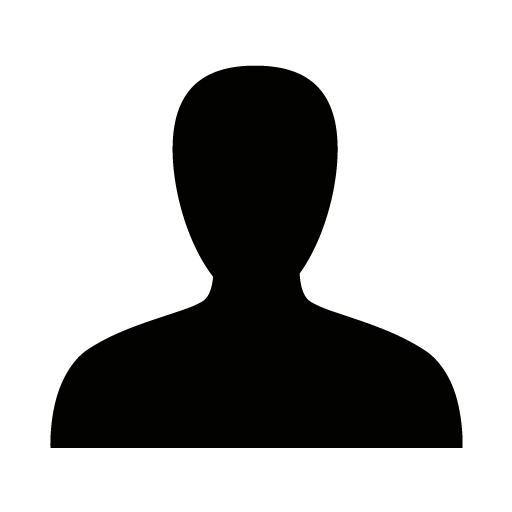
Increasing the concentration of dopants can be accomplished by enhancing the material's stability through the incorporation of a broader range of constituent oxides. This explains the recent development of high entropy/multi-constituent oxides, driven by the interest generated from research on high entropy alloys [1]. The enhancement of dopant concentration can be especially important in the field of protonics[2]. In the past four years we have studied a series of multiconstituent oxides and showed that they exhibit protonic conductivity or mixed ionic-electronic conductivity[3,4]. However, even if this group of oxides can potentially revolutionize the applied ceramics field there is a lot of questions stemming from high complexity of this vast materials group. From structural aspects, through electrochemical performance, to thermochemical properties, the abundance of constituents complicates the comprehension of specific properties that at the end will affect the performance of future devices. Our research is dedicated to unraveling the fundamental phenomena that govern the properties of this class of oxides.
In this talk I will focus on the high entropy and medium entropy oxides which stems from well characterized low entropy end-members including barium zirconate-barium cerate system. The presentation will cover properties such as electrical conductivity, water absorption, and thermodynamic stability. All this topics are lively involved in determination if so called high entropy oxides are indeed a new promising materials for applications in protonic ceramic electrochemical cells.
Gielgud-O1
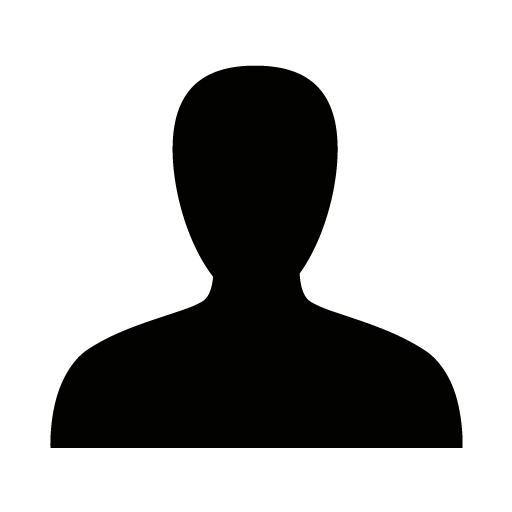
The global demand for sustainable and reliable energy sources has led to increased interest in technologies such as solid oxide electrochemical cells (SOECs), which reversibly generate electricity and hydrogen through chemical reactions. However, SOECs require high operating temperatures, posing material and cost challenges. Protonic ceramic electrochemical cells (PCECs), on the other hand, utilize protons as charge carriers and operate at lower temperatures (below 600°C) with higher efficiency due to their low activation energy. So far, triple ionic-and-electronic conductors (TIECs) have been extensively investigated for PCECs, introducing, and enhancing proton conductivity to the oxygen electrode, providing a larger space of active reaction sites for electrochemical reactions in the cell; however, challenges such as A-site cation segregation or inadequate chemical compatibility found in highly active Co-based materials still need to be addressed for practical application. Recently, high-entropy materials have attracted attention to address these issues, exhibiting thermodynamically stable properties at high temperatures results from their high configurational entropy of the material. Here, we present recent progress in the development of high-entropy double perovskite oxide (HEDPO) oxygen electrodes for PCECs, incorporating high configurational entropy in the A-site of the structure to achieve superior material compatibility and stability while maintaining excellent electrocatalytic activity. This work paves the way towards robust and innovative oxygen electrodes for PCEC applications, contributing to the advancement of sustainable energy solutions.
Gielgud-O2
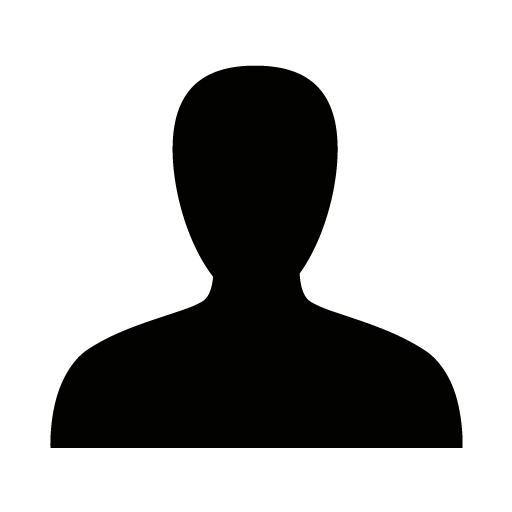
Barium lanthanide cobaltites with perovskite structure have been studied as potential electrodes for electrochemical cells due to their mixed oxygen ionic – electronic conductivity[1]. More recently[2,3], protonic defect formation has been shown in some of these oxides. Moreover, our previous studies have shown that compositional complexity induces changes in both the structure and transport properties of this system[4]. Therefore, we decided to take a step further and leverage the emerging field of high entropy oxides. In these materials, compositional complexity is taken to the extreme by introducing up to a dozen (or even more) cations into one sublattice[5]. This approach allows for the discovery of new material properties and the enhancement of features such as electronic and/or ionic conductivities
This talk will focus on how structure, chemical composition and electronic states affect protonic defect formation and total conductivity of BaLnCo2Fe1-xO6-δ. The study explores first simple compositions with one or two different lanthanides on the A-site and then introduces up to 12 different elements in one sublattice in equimolar proportions (e.g. BaLa1/12Ce1/12Pr1/12 Nd1/12 Sm1/12 Eu1/12 Gd1/12Tb1/12Dy1/12Ho1/12Er1/12Tm1/12Co2O6-δ). We evaluate changes in structure, total conductivity, Seebeck coefficient and water uptake occurring as an effect of introducing multiple elements in one sublattice. For selected compositions partial protonic conductivity is measured using a modified Hebb-Wagner DC polarization method.
Fleming-K1
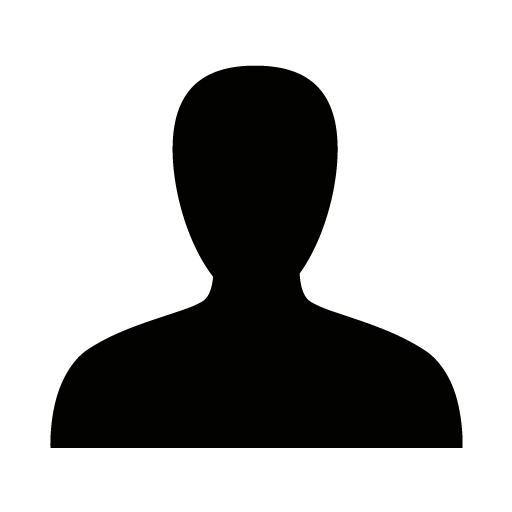
For the past decades, extensive efforts have been placed in improving the performance of the layered compounds for cathodes such as by compositional tuning and structural modifications. One of the notable approaches in recent years is to adopt excess amounts of lithium-ions in the layered materials, which surprisingly revealed that the specific capacity can be boosted in the layered cathodes via the shift from the conventional cationic redox reaction relying on transition metals (Co, Ni and Mn) to the cumulative cationic and anionic (oxygen) redox reaction. In this journey to explore the ‘lithium-excess layered cathodes’, various new findings have been being disclosed. In this talk, I will present our recent understandings on these materials with respect to the lithium insertion mechanism that differs from what have been observed in conventional layered materials and the effect of the layered stacking sequences. We report a novel series of lithium-rich layered oxides (LRLO) with O2-type oxygen stacking, which effectively address the persistent challenges associated with the voltage and structural deterioration observed in conventional LRLO cathodes. Our newly developed LRLO cathodes exhibit minimal structural disordering even with the anionic redox participation as much as ~ 90 % (based on the nominal composition corresponding to ~ 250 mAh g-1), while maintaining a stable discharge voltage.
Fleming-O1
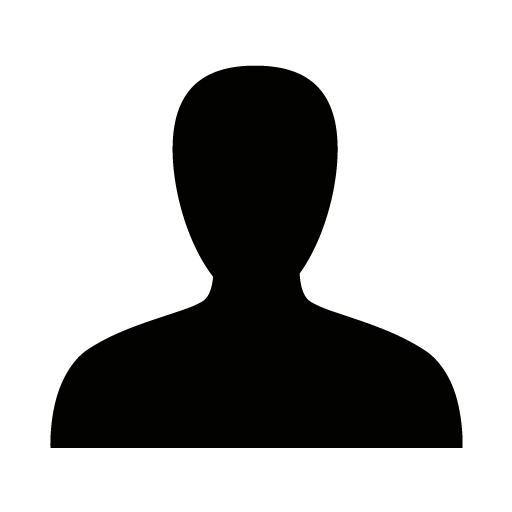
Electrochemical energy storage relies on electrode materials facilitating ion and electron transport. In solid-state batteries, the electrode architecture typically comprises multi-phase composites, including mixed ion-electron conductors (e.g., LixNi8Co1Mn1O2 (NCM)), solid electrolytes (e.g., Li7La3Zr2O12 (LLZO)), and electronically conductive carbon (e.g., carbon black). Charge transport in such heterogeneous systems is intricate and is often erroneously analyzed using traditional theories developed for homogeneous electrodes, leading to a misunderstanding of electrode kinetics in real battery cells.
In this contribution, we present a comprehensive treatment for characterizing the kinetics of composite electrodes. Our approach considers not only the composite's microstructure but also the heterointerfaces between its constituents, significantly influencing electrical and chemical transport phenomena in composites. Employing electrochemical impedance spectroscopy, we identify non-ideal spectra and the corresponding time constants of heterogeneous systems using a generalized transmission line model. Furthermore, to capture the morphological nature of composites, we reconstruct the 3D structure of practical electrodes consisting of NCM, LLZO, and carbon black through transmission X-ray microscopy (TXM). By visualizing concentration propagation during lithium insertion, we unveil the critical roles of size, shape, coating, and contact effects in composites, providing guidelines for rationally designing electrode architectures in solid-state batteries. The treatment developed herein sheds light on understanding and predicting the behaviors of charge carriers in complex electrochemical systems.
Fleming-O2
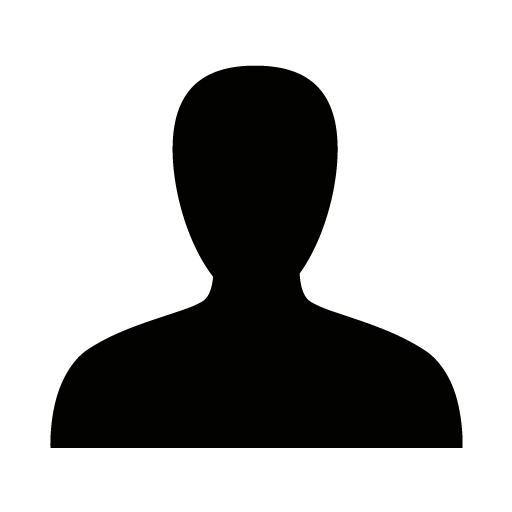
All-solid-state lithium-ion batteries (ASSBs) using inorganic electrolytes (SEs) are promising candidates for next-generation batteries because of their improved safety and high energy density. However, bringing out the performance of ASSBs beyond conventional lithium-ion batteries (LIBs) is still a challenge. The ASSBs with high power density will be achieved by optimizing ionic and electronic conduction properties in the electrode composites[1], which depend on the microstructural properties of the composites. The relationship between the conduction and microstructural properties is generally evaluated by macroscopic parameters based on the tortuosity factor and effective conductivity[2]. However, the relationship should also be evaluated from the microscopic conduction properties of the electrode composites for ASSBs, which is not well understood so far.
The scanning spreading resistance microscopy (SSRM) technique directly measures current distributions at the sample surfaces in the nanoscale. Otoyama et al. analyzed the local resistance using SSRM to demonstrate the presence of electrically isolated cathode active material (CAM) particles in ASSBs after charging, providing information on the electronic contact of CAMs[3]. The local resistance analysis using SSRM is an effective evaluation technique for optimizing the electrode design for high-performance ASSBs.
Herein, we investigated the microscopic electronic conduction properties of cathode composites blended with LiNbO3-coated LiNi0.5Co0.2Mn0.3O2 (NCM) and sulfide SEs at a volume ratio of 22:78, 34:66, 45:55, and 66:34 using the SSRM technique. Some of the NCM domains in the 22NCM-78SE cathode composites were electrically isolated. The presence of the isolated NCM leads to a significant capacity loss. In 45NCM-55SE and 34NCM-66SE cathode composites, all the NCM particles were electrically connected to a current collector. These cathode composites showed bimodal resistance distributions in the NCM domains. The dispersion of the local resistance indicated the existence of NCM particles with poor electronic transport pathways, causing still limited capacity (105 mAh g−1). The 66NCM-34SE cathode composite exhibited unimodal resistance distribution in the NCM domains. This microscopic conduction should result in a homogeneous current distribution, thus allowing for sufficient utilization of NCM (150 mAh g−1). To achieve high-performance ASSBs, all NCM particles in cathode composites are required to be connected to the current collector in contact with each other. The analysis of microscopic conduction properties using the SSRM technique provides insight into an optimized microstructure design to realize ASSBs with high power density.
Fleming-O3
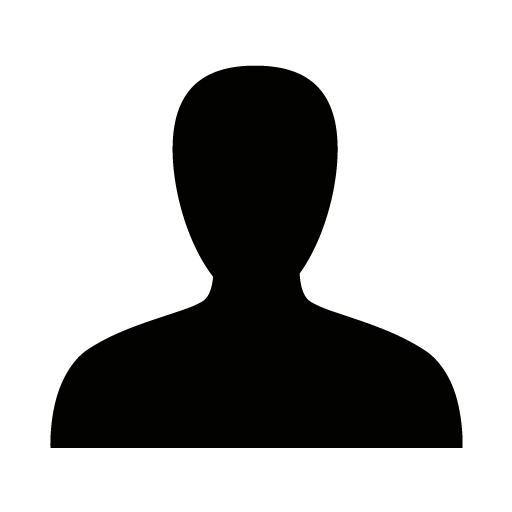
Experimental studies have revealed a kinetic plateau at 3.5 V in the composition-voltage curve of LiNiO2 during slow charge/discharge cycling, such as C/20. However, this plateau and its associated capacity diminish at higher rates. Interestingly, even slight temperature variations have a significant impact on this kinetic plateau: cycling LiNiO2 at 45 °C instead of 25 °C results in the recovery of a substantial capacity, primarily at 3.5 V. Additionally, slow discharge at low voltage (a potentiostatic process at 2 V for 1 month) can also restore the lost capacity.
Over the years, various explanations have been proposed to account for the hindered kinetics plateau and the corresponding pseudo-irreversible capacity. In our study, we demonstrate that the presence of excess Ni in LiNiO2 significantly slows down the material's kinetics. This effect can be attributed to two critical mechanisms.
Firstly, the excess Ni in the Li layer exerts an attractive force on Li vacancies, reducing their energy compared to defect-free regions and effectively transforming excess Ni into a sink for lithium vacancies. This attractive force arises from the relatively positive charge of Ni and the strain it induces in the material. It extends across a radius of two lattice sites, substantially reducing the barrier for Li vacancies to approach the defect. Similar arguments apply to divacancies, which are predominantly pinned to excess Ni in the form of split divacancies. At low temperatures, trapping leads to lower diffusion coefficients compared to values extrapolated from higher temperatures. This finding expands upon Delmas' popular hypothesis, which has been the prevailing explanation for the difficulty in reintercalating Li after the first cycle, but challenges the notion of excess Ni oxidation or any local lattice shrinkage of the Li layers surrounding the defect.
Secondly, the presence of excess Ni hinders Li migration due to its inherent immobility and inability to undergo site exchange with adjacent Li vacancies. These findings highlight the crucial role of excess Ni in influencing material kinetics by simultaneously reducing the number of charge carriers and increasing the tortuosity of diffusion paths.
Fleming-O4
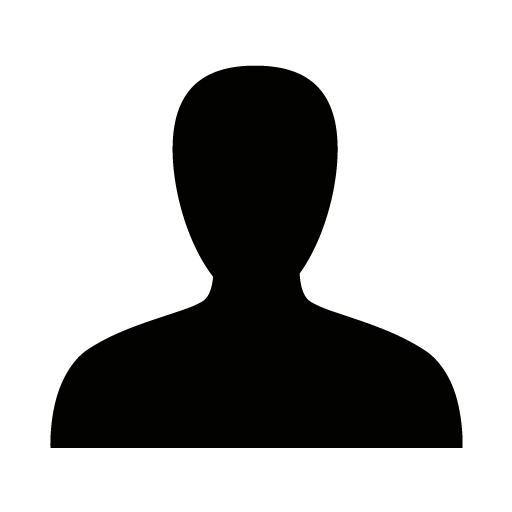
Cation doping have been widely applied for the development of energy storage/conversion materials. However, due to extensive works such as combinatorial synthesis and data-driven material explorations, the effectiveness of cation doping is reaching the limitations. Instead of cation doping, anion doping is one of the most promising new strategies for energy material development, although tuning of anion composition is technically difficult due to mismatch of ionic size and volatility. To overcome such difficulties, we recently develop electrochemical fluorine doping reactor and succeeded to introduce F defect into the oxide host structure [1]. In this work, fluorine doping into an oxide-based lithium-ion battery cathode is examined by using our electrochemical F doping reactor, to understand influences of anion defect on battery performance.
LiMn2O4 was synthesized by solid state reaction method from Li2CO3 and Mn2O3. For F-doping, oxygen-deficient LiMn2O4-d was prepared by annealing 1%O2 at 700oC. F-doping reactor was composed of Pt/La0.9Ba0.1F2.9/PbF2-Pb, and the oxygen-deficient LiMn2O4-d was placed onto the Pt current collector. The reactor was uniaxially pressed and heated at 250oC, and then fluorine was electrochemically introduced the small compartment where LiMn2O4-d exist. The amount of supplied F was controlled by electric charge passing through the reactor. F-doped LiMn2O4 was characterized by XRD, XPS, TOF-SIMS. The composite cathode was prepared from the cathode active maerials:PVDF:Carbon = 70:10:20, and the structure of the battery test cell was the composite cathode/1M LiPF6 EC:DMC=1:1/Li metal. The battery performance was evaluated by HJ1001SD8 (Meiden Hokuto Corp.) at 25oC.
After F-doping treatment, no impurity phase was observed, and the F-doped LiMn2O4 maintained the original spinel structure. Clear F-1s peak was detected from F-doped LiMn2O4 by XPS and its intensity increases with increase the amount of supplied F. The uniformity of the fluorine distribution in the LiMn2O4 particle was checked by TOF-SIMS. While F intensity of the particle surface was slightly higher than that of the bulk part, clear F signal was confirmed at the deep inside of the particle. This means our F pumping treatment successfully introduce fluorine defect not only on the surface but also into the particle inside.
To understand the influence of anion defect species, oxygen vacancy and fluorine defect in this study, charge discharge measurement was performed on the oxygen-stoichiometric LiMn2O4, the oxygen-deficient LiMn2O4-d and F-doped LiMn2O4. Among them, the oxygen-stoichiometric LiMn2O4 showed the best capacity retention upon charge discharge cycles. The oxygen-deficient LiMn2O4-d showed significant capacity degradation upon cycles, suggesting that oxygen vacancy significantly degrade battery performance [2]. On the contrary, F-doped LiMn2O4 showed better cycle stability than the oxygen-deficient LiMn2O4-d, suggesting that F defect improve the battery performance. These indicate suitable tuning of anion defect structure can be a promising new strategy for battery material development.
Fleming-O5
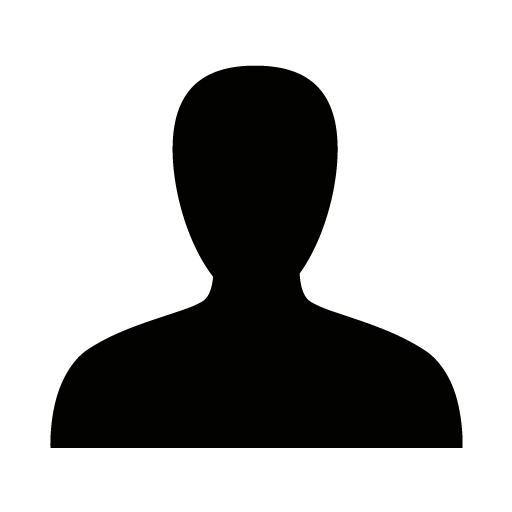
Metal-organic frameworks (MOFs) constitute a class of porous materials comprising inorganic metal nodes and organic ligands. Due to their designable chemical and structural diversity, MOFs have recently demonstrated high tunability in controlling the functionalities of electrodes, thereby paving the way for the development of fast-charging batteries. In this contribution, we present a novel class of MOFs based on the ligand bis(2-hydroxyethyl) terephthalate (BHET), exhibiting an anomalous behavior in lithium storage in response to insertion reactions. In contrast to conventional battery materials, which often suffer from degradation in storage capacity, BHET-based MOFs exhibit a distinctive behavior wherein significant growth in charge storage is observed even after hundreds of cycles of lithium insertion and extraction. This anomalous phenomenon of charge storage indicates an electrochemically self-activated reaction occurring in BHET-based MOF electrodes.
X-ray diffraction characterization reveals a dramatic structural transformation, turning the MOF structure from well-crystalline to amorphous during (de)lithiation processes. Furthermore, an investigation using transmission X-ray microscopy (TXM) demonstrates the evolution of pore construction during battery cycling. These structural characterizations, in conjunction with the redox of metal node ions studied by X-ray absorption spectroscopy (XAS), represent that the microstructural transformation of BHET-based MOFs drives the self-activation reaction, leading to the anomalous growth of charge storage capacity within each cycle. Additionally, we observe a transition in the storage mechanism of BHET-based MOF electrodes, initially involving bulk-type intercalation reaction and eventually shifting to an interface-type pseudocapacitive storage mechanism. These findings not only elucidate the complex storage mechanism in MOF-based materials but also shed light on the design of high-performance and long-lasting batteries.
Westminster-K1
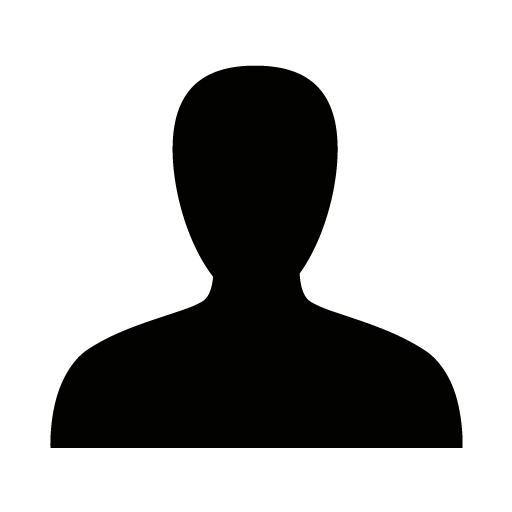
Development of new oxide ion conductor is important subject [1-3]. Recently new bismuth containing phases such as Dion-Jacobson phase CsBi2Ti2NbO10-δ or Sillén-Aurivillius phase, Bi3.9Sr0.1NbO8-δCl were reported as new oxide-ion conductors reaching log(σ /S∙cm-1) = 8.9 10-2 at1174 K and log(σ /S∙cm-1) = 2.99 10-2 at 873 K, respectively [1-2]. It was previously reported that the halide layer in the bismuth niobium oxychloride has a positive stabilizing effect and increases the stability of the material [1]. In this study, the conductivity of PbBiO2Cl, Sillén phase, a layered oxychloride material, explore substitution possibilities of Pb and Bi in the (PbBiO2)+ layer with iso- and aliovalent cations for increasing the oxide ion conductivity through introduction of interstitial oxygen or oxygen vacancies were measured.
Various cation doped PbBiO2Cl were successfully prepared and substitution of bismuth with an alkaline earth and an isovalent cation simultaneously (Ca2+ and Ga3+) is the most effective for increasing the conductivity and stability of PbBiO2Cl. The optimized dopant values were found to be 25mol% Ca and 10mol% Ga, PbBi0.65Ca0.25Ga0.1O2Cl. From electromotive force on O2-N2 cell and DC-polarisation measurements, oxide ion is not the major conducting spices which is estimated to be 20% or less of the total observed conduction, but the chlorine ion seems to be major charge carrier of which transport number is estimated to be higher than 40%. Consequently, this study reveals that doped PbBiO2Cl is a mixed anion conductor of Cl- and oxide ion, and small hole conduction. The oxide ion and Cl- conduction may occur in the bismuth-oxide [Bi2O2]2+ or [PbBiO2]+ layers and Cl layer in the Sillén and Sillén-Aurivillius structure, respectively.
Westminster-O1
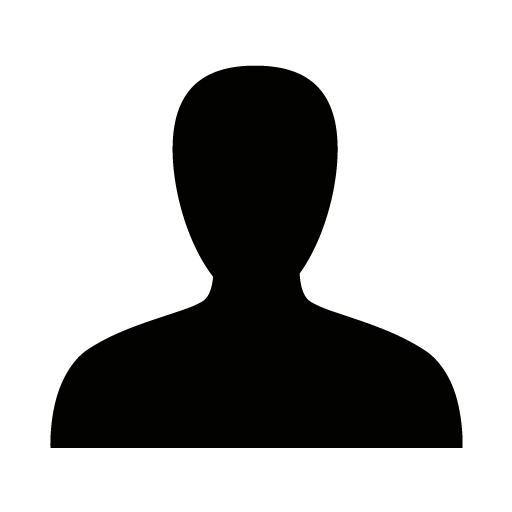
Hydrogen-related technologies are gaining increased attention as a solution to achieve carbon neutrality. Solid oxide fuel cells (SOFCs) can generate electricity with high efficiency (about 60-80%) by reacting hydrogen and oxygen at high temperatures (generally over 800℃) without using precious metal catalysts. Low- and medium-temperature fuel cells that operate in the 500~800℃ range are presently being studied due to the rapid deterioration and the high cost of the specialized stack and system materials needed to enable sustained operation at high temperatures. While lower temperature operation is highly desired, the oxygen reduction reaction (ORR) in the cathode slows down when the operating temperature is lowered. Therefore, there is a need to develop cathode materials with high efficiency in the intermediate- and low-temperature range [1, 2].
Oxygen vacancies promote ORR by providing an oxygen path in the crystal structure and enhancing the oxygen ion conductivity. Charge transfer to the adsorbed oxygen ions is caused by the electronic structure and promotes ORR. The charge transfer energy along with oxygen vacancy concentration is an important screening metric that can be used to identify promising perovskite oxides with potentially improved electrocatalytic activity [3, 4]. Recently, the charge transfer energy has received increasing attention because it directly affects the charge transfer between adsorbed oxygen and the cathode surface, which is the rate-determining step of ORR.
We investigated the ORR reaction mechanism and electrochemical properties of PrBa0.9A0.1Co1.9Cu0.1O5+δ (PBACCu, A=Ca, Nd) from the perspective of valence electronic structure with those metrics. Each composition has a tetragonal crystal structure, and the lattice shrinks with Ca and Nd doping, which have smaller ionic radii than Ba. XPS analysis reveals an average oxidation number of 3.3 for both PBCaCCu and PBCCu. However, a charge disproportionation (Co3+ → Co2+ + Co4+) was observed. The average oxidation number of PBNdCCu decreased to 3.19, indicating the formation of additional oxygen vacancies. Deconvolution of the O 1s XPS spectrum revealed a decrease in lattice oxygen for the Ca and Nd doped compositions, indicating that A site doping enhances covalency. The oxygen vacancy peak in PBNdCCu increased from 54.27% to 62.68%, consistent with the average oxidation number analysis of the metal ions. Additionally, the B-O charge transfer energy was calculated from the XPS valence band spectra, yielding -3.60 eV for PBCCu, -3.45 eV for PBCaCCu, and -4.00 eV for PBNdCCu. Symmetric cells of all three electrode materials were examined by electrochemical impedance spectroscopy (EIS). The EIS results indicate that PBCaCCu has the smallest polarization resistance (0.062 Ω cm2) compared to PBCCu (0.113 Ω cm2) and PBNdCCu (0.101 Ω cm2) at 700°C, consistent with its lower charge-transfer energy. The effects of oxygen vacancy and charge transfer energy on the oxygen reduction reaction mechanism are further illuminated with the use of DRT analysis.
Westminster-O2
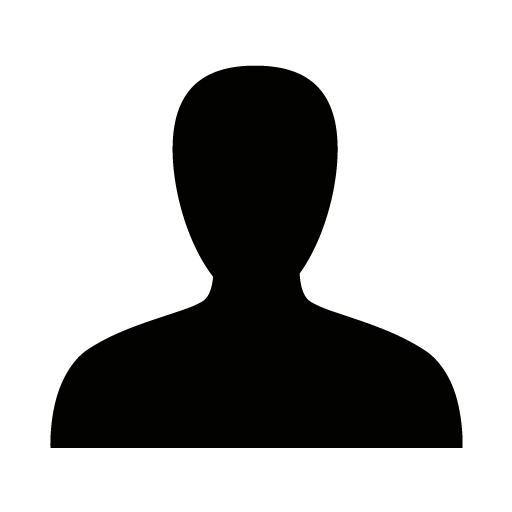
Doped CeO2-based electrodes emerge as an alternative to state-of-the-art Ni-based cermets electrodes for Intermediate Temperature- Solid Oxide Cells (IT-SOCs), offering mixed ionic-electronic conductivity (MIEC) which combined with Ni-Fe alloys can lead to carbon-tolerant electrodes. Sm co-doping Ce0.9Zr0.1O2 has shown to be a great strategy to improve the electrocatalytic activity and conductivity of electrodes1. Near-Ambient Pressure X-Ray Photoelectron Spectroscopy (NAP-XPS) and Near Edge X-ray Absorption Fine Structures (NEXAFS) combined with electrochemical techniques were carried out at synchrotron facilities (ALBA and Diamond Light Sources) under operando conditions to identify the active species leading to the increased performance of the cells and to distinguish the role in the cermet activity of Sm co-doping from the contribution of Ni-Fe bimetallic alloys. For instance, NAP-XPS results show that an excellent carbon-tolerant electrode material was engineered. Ce, Ni, and Fe active species dictate the electrochemical response of the cell, and we observed that the redox properties of Fe and Ce, resulting from the applied potential, have the greatest influence. Also, the surface chemistry is restored when no potential is applied. Moreover, it has been observed that Fe predominantly reacts with any sulfur present in the fuel, apparently preventing the deactivation of Ni by sulfur poisoning. Thus, the activation of CO2 towards CO production in a H2/CO2 gas mixture was also demonstrated. Furthermore, X-ray Diffraction technique (XRD) were performed under operando conditions (at Bessy II Light Source) and demonstrated that the crystalline structure remains stable and unchanged throughout the operation.
Westminster-O3
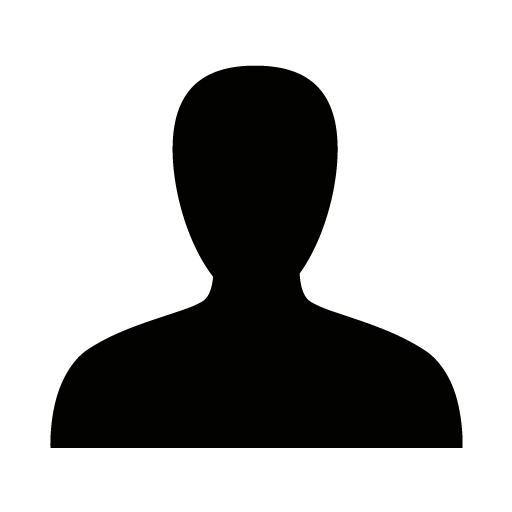
Hydrogen has emerged in recent years as a source of clean energy production as long as it is not produced with the emission of carbon dioxide. One of the most promising options is the use of electrolyzers in combination with renewable electricity sources to produce hydrogen cleanly. However, the efficiency is still low, and the development of new materials is necessary to make these devices competitive.
The objective of this work is to characterize the SrFe1-xSbxO3-δ solid solution in order to design a functional material for use as an air electrode in solid oxide electrolyzers for the production of green hydrogen.
SrFe1-xSbxO3-δ perovskites-like materials (x = 0.05-0.20) were prepared by solid-state route in air at 1350°C. XRD results showed the formation of single-phase samples with tetragonal structure for the compositions range x = 0.05-0.10, whereas higher antimony contents (x ≥ 0.15) produce single-phase samples with cubic symmetry. The characterization of materials included microstructural studies (SEM/EDS), measurements of the Area of Specific Resistance (ASR) of a 30-70wt% of CGO-material composite in air, electrical conductivity as function of temperature at different oxygen partial pressures, determination of oxygen content, compositional analysis by XPS and structural characterization by SAED and HREM. Initially, for x = 0.05 to 0.10, ASR values (Figure 1) increase with Sb content. However, for higher Sb content (x ≥ 0.15) lower polarization resistances are obtained. Notably, for x = 0.2 the ASR value at 700°C is as low as 0.32 Ω cm2 which is competitive for real devices
Westminster-O4
Juan Bisquert (pHD Universitat de València, 1991) is a Professor of applied physics at Universitat Jaume I de Castelló, Spain. He is the director of the Institute of Advanced Materials at UJI. He authored 360 peer reviewed papers, and a series of books including . Physics of Solar Cells: Perovskites, Organics, and Photovoltaics Fundamentals (CRC Press). His h-index 95, and is currently a Senior Editor of the Journal of Physical Chemistry Letters. He conducts experimental and theoretical research on materials and devices for production and storage of clean energies. His main topics of interest are materials and processes in perovskite solar cells and solar fuel production. He has developed the application of measurement techniques and physical modeling of nanostructured energy devices, that relate the device operation with the elementary steps that take place at the nanoscale dimension: charge transfer, carrier transport, chemical reaction, etc., especially in the field of impedance spectroscopy, as well as general device models. He has been distinguished in the 2014-2019 list of ISI Highly Cited Researchers.
Hysteresis, observed in the current-voltage characteristics of electronic and ionic devices, is a phenomenon in which the shape of the curve is influenced by the speed of measurement. This phenomenon is a result of internal processes that introduce a time delay in the response to external stimuli, causing measurements to depend on past disturbances. Hysteresis and time delay effects find important applications in devices that are explored for resistive switching and neuromorphic computation, such as halide perovskite and organic memristors and transistors for synapses and neurons. Hysteresis and memory effect are also a central component of the electrochemical response of biological protein channels and neurons.
Here we show a classification of various manifestations of hysteresis by identifying common elements. Our approach involves examining hysteresis from multiple perspectives, employing simplified models that capture fundamental response patterns. We investigate system behavior using techniques like linear sweep voltammetry, impedance spectroscopy, and the analysis of transient currents resulting from small voltage steps. Our analysis uncovers two primary types of hysteresis, characterized by how current responds to rapid sweep rates: capacitive hysteresis and inductive hysteresis. These terms correspond to the dominant component in the equivalent circuit, which governs the transient time response.
Westminster-O5
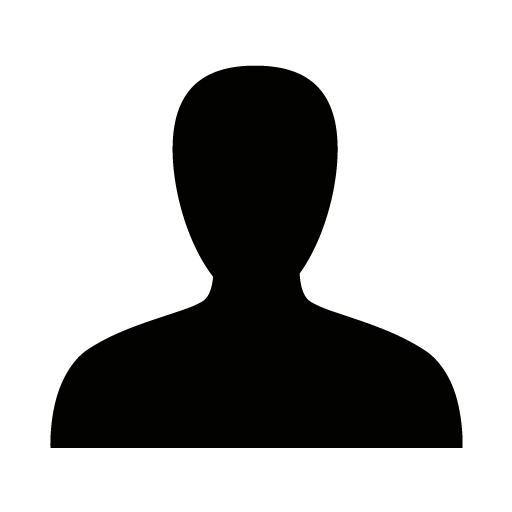
Redox processes in transition metal oxides have been in the focus of solid-state research for decades, given their substantial potential for applications in the fields of sensorics, information technology, superconductivity, and energy conversion. In this context, strontium titanate has become one of the most intensively-investigated solid oxide with perovskite structure and mixed ionic-electronic conductivity. Despite of these considerable research activities, the role of extended defects such as dislocations and grain boundaries in the electronic and ionic transport remains under debate. It is well known that the oxygen nonstoichiometry determines the amount of electronic charge carriers allowing for a control of the electronic conductivity via self-doping by oxygen vacancies upon reduction and oxidation. Assuming a homogeneous bulk phase, this effect can be modelled using the concepts of point defect chemistry in good agreement with macroscopic experimental data [1]. When having a more detailed look on the crystal’s properties on the nanoscale, however, it appears that the redox phenomena are far more complex than previously thought. We present experimental investigations on single crystals, bicrystals and ceramics using high-resolution imaging techniques such as local-conductivity atomic force microscopy (LC-AFM), Kelvin probe force microscopy (KPFM), and scanning nearfield optical microscopy (SNOM), which reveal that dislocations are easy reduction sites where oxygen vacancies are preferentially generated. In turn, filaments with high electronic conductivity evolve around the dislocations in the originally insulating matrix and act as nanoscale short circuits [2]. As dislocations form a three-dimensional network in the surface layer and along of inner surfaces such as grain boundaries, the macroscopic properties of strontium titanate can be turned from insulating to metallic and even to superconducting behaviour. This transition takes place at a very low global oxygen vacancy concentration thus reaching a state that is far outside the prediction of homogeneous defect chemistry. Since there is an attractive interaction between dislocations and oxygen vacancies, the nanoscale conducting filaments can even be manipulated mechanically at room temperature [3]. As dislocations are intrinsically present in real crystals and ceramics, our findings not only can explain failure mechanisms in solid oxide electrolytes, but also challenge traditional models describing the mechanisms of electronic transport and superconductivity in self-doped transition metal oxides.
St.James-K1
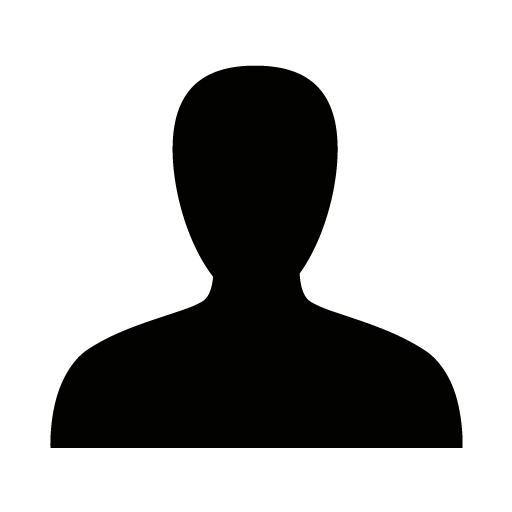
Proton ceramic electrochemical cells (PCECs) comprising fuel cells (PCFCs), electrolysers (PCEs), and reactors (PCERs) require well-conducting electrolytes of mechanical and thermochemical stability, but even more so electrodes with sufficient electrocatalytic activity at moderate temperatures. Oxide positrodes for PCFCs and PCEs are particularly challenging due to limited surface catalytic activity for the oxygen redox reaction and solubility of protons. Current research aims to improve these by, e.g., exsolution of catalytic nanoparticles and optimisation of hydration thermodynamics, in addition to microstructure. Interpretation of experimental polarisation results and degradation phenomena requires physicochemical understanding of the reaction mechanisms and microstructural paths and application of appropriate mathematical models, a field underdeveloped for solid oxide electrochemical cells (SOECs) in general and for PCECs in particular.
The polarization processes at solid-state electrodes comprise space charge layer depletion resistance, charge transfer between the electrolyte and electrode phases, and mass transfer in and on the electrode material. The latter comprises diffusion in and on the solid electrode material and in the gas phase as well as surface reaction kinetics. For PCECs, the processes on Ni metal negatrodes involves diffusion of dissociated atomic hydrogen,[1] while the processes on mixed proton-electron conducting oxide positrodes involves proton-proton charge transfer, proton diffusion, and the surface oxygen-steam electron transfer redox-reaction.
Electrochemical impedance spectroscopy (EIS) enables separation of the different polarisation processes by their capacitances. The proton-proton charge transfer may be expected to follow Butler-Volmer (BV) type kinetics. The mass transfer polarisation of porous mixed conducting electrodes involves coupled diffusion, reaction kinetics, and chemical capacitance that in the simplest case can be modelled as a Gerischer impedance when it polarises the BV charge transfer. This approach for mixed proton-electron conducting (MPEC) electrodes for PCECs follows essentially all the principles for corresponding mixed ion-electron conducting (MIEC) electrodes for SOECs laid down in what we may call the Adler-Lane-Steel (ALS) model. [2,3] Individual polarisation resistances measured by EIS under DC bias can be integrated over current to obtain individual overpotential-current curves and help to identify processes and predicting behaviours under operation in fuel cell or electrolyser mode, also involving complications due to competing oxide ion and p- and n-type electronic conduction.[4]
St.James-O1
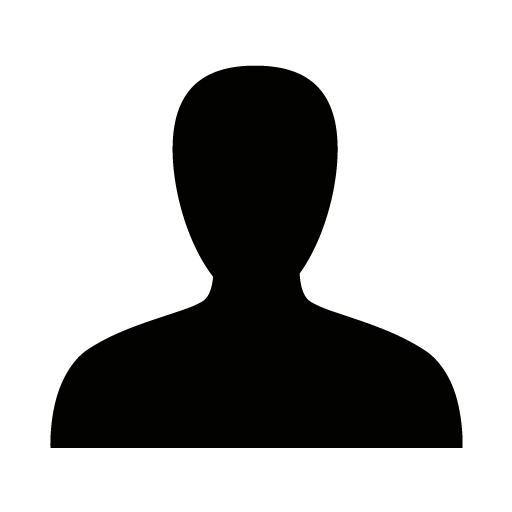
Effect of hydration on electrical and electromechanical properties of lanthanum-cerium oxides
Or Ben Zion1, Tahel Malka1, David Ehre1, Isaac Abrahams2 and Igor Lubomirsky1
1Department of Molecular Chemistry and Materials Science, Weizmann Institute of Science, Rehovot 7610001, Israel
2 Department of Chemistry, Queen Mary University of London, Mile End Road, London E1 4NS, UK.
Keywords: Lanthanum cerium oxide; Lanthanum hydroxide; Proton conductivity; Ceramics; Grain boundaries
In recent years, there has been a growing interest in the pursuit of efficient, clean, and environmentally friendly energy sources. Fuel cells have emerged as a promising technology, allowing the conversion of the chemical energy present in fuel gases into electrical work. Solid oxide fuel cells, utilizing ion-conducting ceramics as electrolytes, have been at the forefront of these developments. However, a significant drawback has been the high operational temperatures typically required, ranging from 300°C to 900°C, due to the thermally activated nature of ion conduction in ceramics.
All solid oxide fuel cells (SOFCs) and electrolyzers (SOEs) operating above 300 °C demonstrate rapid electrode kinetics, especially those based on proton-conducting systems, but are limited in their long-term stability due to thermal stress. Ceramic devices operating between 150-250 °C could enjoy rapid electrode kinetics, even without Pt-based catalysts, and would avoid large thermal stress. However, proton conducting ceramics showing appreciable conductivity in this temperature range have yet to be identified. Developing such a material could revolutionize the field of renewable energy.
Hydrated rare earth oxides, known for their chemical and thermal stability, have emerged as potential low-temperature proton conducting ceramics. Our study of La(OH)3, a material insoluble in water and chemically stable in the presence of carbon dioxide, revealed that the dominant contribution to the conductivity of La(OH)3 below 200 °C is protonic. However, it is not sufficiently high for practical applications. The grain boundaries in La(OH)3 ceramics are non-blocking for proton transport, inspiring further investigation.
We have investigated the partial substitution of La3+ by a tetravalent cation, like Ce4+, to increase the oxygen to hydrogen ratio. We have found that ceramics of LaxCe1-xO2-x/2 (LCO) with x > 0.5 decompose to La(OH)3 and CeO2 upon contact with water or water vapor, limiting the search range to x ≤ 0.5.
We have developed a hydration protocol for LCO50 ceramics, leading to ≈7.5±1% of hydration with respect to the theoretical limit. The hydration results in a 0.17% lattice expansion due to water incorporation and a large increase in electrical conductivity (Figure 1). Further hydration results in the mechanical disintegration of the ceramic pellets, prompting the investigation of LCO with x < 0.5.
No evidence for water incorporation for x = 0.2 and x = 0.4 compositions was found under any conditions. Hydration of LCO45 at 200 °C for 48 hours in steam causes fragmentation of the ceramic pellet. However, as determined by thermogravimetric analysis, the replacement of steam by water-saturated nitrogen (25°C) results in 17% of the oxygen vacancies being filled up, expansion of the lattice by 0.18%, an increase in electrical conductivity, and a decrease in activation energy.
We expect that further work will lead to a viable proton conducting ceramics suitable for use a bulk and micro-fabrication-compatible thin films.
St.James-O2
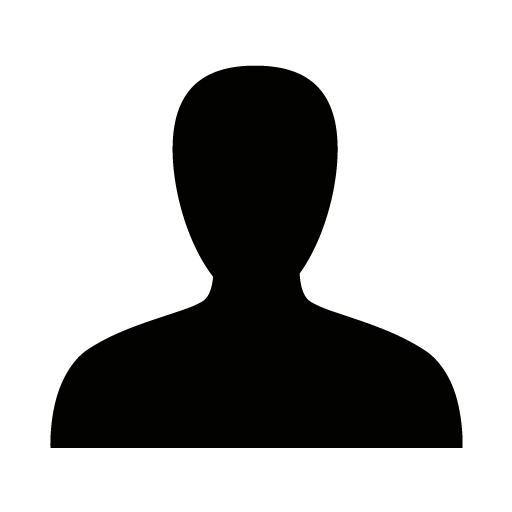
Proton-conducting oxides can be utilized in numerous sustainable devices, such as protonic fuel cells [1] and low-power electronics [2]. In order to optimize materials and meet specifications for commercial viability, better understanding of transport mechanisms is required. While electronic and structural descriptors have been found for predicting proton conductivity [3], there are knowledge gaps in the field on the effects of lattice dynamics.
For ternary oxides in particular, protons stay localized to oxide-ions and obey the Grotthuss mechanism [4], where the energy barrier of proton-hopping is believed to correlate with donor-acceptor spacing [5]. Within this class of proton conductors, lattice dynamical studies have been largely limited to perovskites. For example, shrinking donor-acceptor spacing via bond-angle-bending [6] and octahedral tilting [7] were found to be important for proton-transport in a handful of undoped perovskites. Another study found correlations among doped perovskites between energy barrier and phonon-related quantities such as averaged atomic mass [8]. However, further work is required to elaborate on the specific phonon modes facilitating proton-hopping and to formulate descriptors generalizable across different crystal structures.
Here, we developed a featurization of phonon spectra called thermal O...O fluctuation, which quantifies the flexibility of the oxygen sublattice and correlates with energy barriers of proton-hopping for a variety of ternary oxides. Analysis of this featurization can highlight which modes contribute most to thermal O...O fluctuation, while capturing frequency-dependence with higher weighting for low-energy modes. Therefore, we anticipate that this analysis will identify modes which assist proton-hopping. Applying this to the well-studied perovskite BaZrO3, we extracted the phonon modes dominating thermal fluctuations of nearest-neighbor O...O pairs and found that they align well with the bending and rotational modes identified in literature [6,7]. Applying this featurization further to 77 O...O pairs found in 10 ternary oxides with varied crystal structures, we likewise observed correlations between thermal O…O fluctuation, equilibrium O…O spacing, and calculated energy barriers. These results imply that both static O…O spacing and dynamic fluctuations must be considered to predict proton conductivity in ternary oxides.
St.James-O3
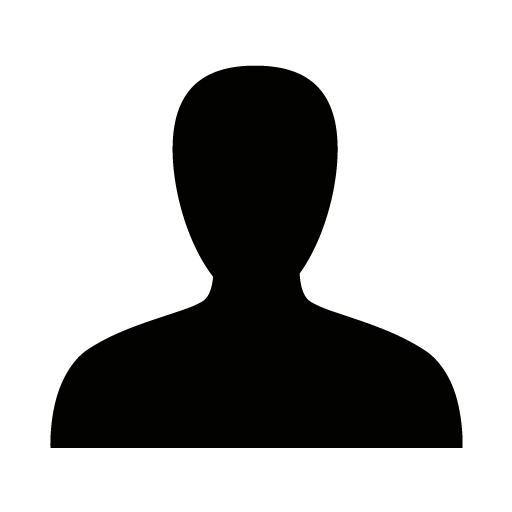
The glass containing transition metal oxides such as Fe2O3, V2O5, WO3, and MoO3 exhibits electronic conduction due to electron hopping between transition metal ions. When alkali oxides such as Li2O, Na2O, and K2O are added to such a glass, its electrical conductivity changes from electronic, mixed ionic and electronic to ionic with increasing alkali oxide content [1]. Because the mixed ionic and electronic conductor has a potential use as an electrode in Li+ and Na+ ion batteries, especially all solid-state batteries [2], the glass containing both transition metal and alkali oxides has recently attracted attention. Protons are monovalent cations that dissolve in glass in large quantities, as do alkali ions. Although the glass is expected to be used as electrodes in fuel cells when the glass is the mixed proton and electron conductor, there have been few studies on the mixed protonic and electronic conduction in glass [3] because it is difficult to obtain glass with high concentration of protons.
In this study, 30HO1/2−6VO5/2−14VO2−50PO5/2 glass was prepared with an expectation that it would be a mixed protonic and electronic conductor by electrochemical substitution of sodium ions with protons (APS) [4] of the 30NaO1/2−10VO5/2−10VO2−50PO5/2 glass. While the 30NaO1/2−10VO5/2−10VO2−50PO5/2 glass was a mixed ionic and electronic conductor, the 30HO1/2−6VO5/2−14VO2−50PO5/2 glass was a pure proton conductor contrary to the expectation. The suppression of the electronic conduction in the 30HO1/2−6VO5/2−14VO2−50PO5/2 glass was investigated on the basis of Mott’s theoretical expression of the small polaron hopping conduction in semiconducting glasses. From the evaluation of the parameters in the theoretical expression and the analysis of the glass structure, the suppression was attributed to the increase in the electron wavefunction decay constant induced by the structural change in the vanadophosphate flamework. Specifically, the vanadium ions exist mainly as the VO4 in the 30NaO1/2−10VO5/2−10VO2−50PO5/2 glass and VO6 in the 30HO1/2−6VO5/2−14VO2−50PO5/2 glass. And the electron wavefunction decay constant for the electron on the vanadium atoms is larger in the VO6 than in the VO4. Therefore, in the 30HO1/2−6VO5/2−14VO2−50PO5/2 glass, where the VO6 with fast electron wavefunction decay is present as the main form of vanadium oxide, the electron wavefunctions between adjacent vanadium ions do not overlap, resulting in a very small electronic conductivity, even though the distance between vanadium ions and the fraction of V4+ ions in the total V atoms are almost the same as in the 30NaO1/2−10VO5/2−10VO2−50PO5/2 glass. The proton conductivity of the 30HO1/2−6VO5/2−14VO2−50PO5/2 glass was as low as 1×10−5 Scm−1 at 300 °C compared to conventional proton-conducting phosphate glasses prepared by using APS due to the low proton mobility resulting from the strong proton trapping by the non-bridging oxygen in VO6.
St.James-O4
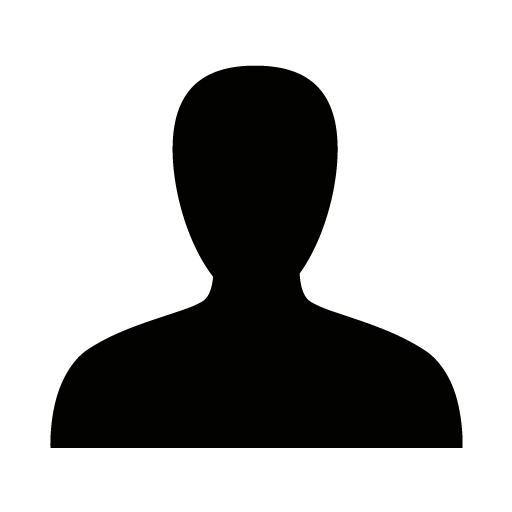
Space charge layers are common at heterogeneous interfaces [1]. Previous investigations [2-4] into the space charge layer (scl) effects at the interface between metal electrodes and proton-conducing electrolytes were predominantly done by the Distribution of Relaxation Times (DRT) method due to the challenge of distinguishing between various transfer processes through impedance spectroscopy. In the present study, the presence of a space charge layer at the interface between Ag electrodes and proton-conducting perovskite electrolytes of BaZr0.9Y0.1O3-δ (BZY10) is evidenced by a well separated semicircle from impedance spectra with characteristic capacitance of ~10-7 F.
The specific conductivities of grain boundaries and the Ag-BZY10 interface space charge layer were calculated on the basis of an ideal brick layer model and measured capacitances. Based on the Mott–Schottky approximation [5], the space charge layer potential, also known as the Schottky potential, was calculated for grain boundaries and the electrode interface vs temperature (300 – 550ºC), pO2 (10-5 – 0.98 atm) and pH2O (0.005 – 0.021 atm).
The Schottky potential at the electrode-electrolyte interface was found to be around 2 times higher than that of the grain boundaries, which for instance may arise from the segregation of protons at the interface in order to reduce the large lattice mismatch between the metal electrode and the perovskite oxide electrolyte. The potential increased with increasing pH2O for both grain boundaries and the Ag-BZY10 interface. The grain boundary potential decreased with increasing temperature, while pO2 had little effect. For the Ag-BZY10 interface potential, there was an apparent interplay between the effects of temperature and pO2. Interpretations of the above phenomena will be proposed and discussed.
Moore-O4
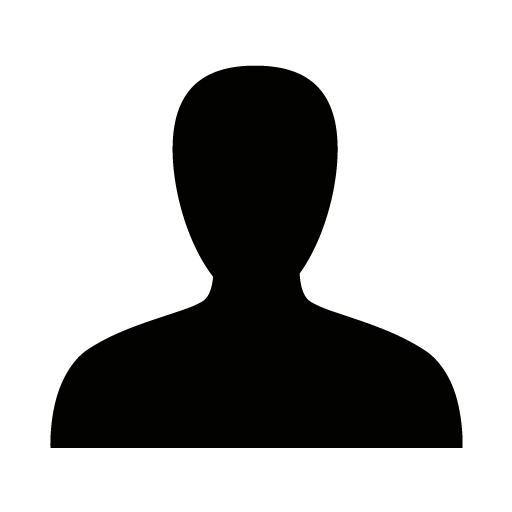
Lithium-ion batteries are one of the most important energy storage devices today, occupying a leading position in the fields of portable electronic products and electric vehicles (EVs). With the rapid expansion of the EV market, people have higher requirements for the cruising range, which forces development of high-energy-density cells, and as consequence, new cathode and anode materials. In terms of cathodes, lithium-rich layered oxide (LLO) materials have attracted increased attention of many researchers, due to their ultra-high theoretical specific capacity and low costs. Such oxides are considered to be one of the most promising candidates for the next-generation cells [1,2]. However, inherent issues, such as low initial Coulombic efficiency (ICE) and voltage attenuation seriously hinder practical application of lithium-rich materials until now. In this regard, researchers are committed to mitigating the first effect through surface coating and bulk doping. Besides the modification on active material itself, introduction of functional additives into an electrolyte is a low-cost and highly targeted method to optimize the performance of LLO electrodes. In fact, electrolyte additives are found to be crucial for optimizing the properties. They can stabilize the electrolyte, remove HF/H2O, adjust the solvent structure, improve flame retardancy, etc. Special electrolyte additives that inhibit TM dissolution and oxygen release are also critical to the electrochemical performance of LLO materials. Also, the composition and structure of cathode-electrolyte interphase (CEI) can be effectively adjusted through the design and use of such additives. Furthermore, multiple additives can be coupled to exhibit synergistic effects in CEI, thereby bestowing in situ its structure with multifunctionality.
In this work, Li1.2Mn0.54Co0.13Ni0.13O2 cathode material was prepared by co-precipitation method, and the influence of various electrolyte additives such as vinylene carbonate (VC), 1,3,2-dioxathiolane-2,2-dioxide (DTD), tris(trimethlysilyl) phosphate (TTSP), tris(trimethlysilyl) phosphite (TTSPi), and lithium 2-trifluoromethyl-4,5-dicyanoimidazole (LiTDi) on the material properties was studied. In order to explore the effect of electrolyte additives on the changes in lattice volume of the electrochemically-active phase during charging and discharging, operando XRD experiments were conducted. A custom-made cell, having a Be window was used for the measurements. The registered charge/discharge curves, obtained using the operando cell, were nearly the same as those obtained using the normal 2032 coin-type half cells. In addition, operando Raman method was used to monitor the vibration of O during the cycling processes of LLO electrodes using different electrolyte additives, demonstrating the role of different additives in inhibiting TM dissolution and oxygen release. Results of XAS measurements at the edge of TM L showed that after 50 cycles at 0.5C the bulk-related signal of the samples with various electrolyte additives did not differ significantly from the initial signal, but there was a significant difference in surface-related signal. Only the samples with TTSP additive exhibited the closest signal to the initial samples, indicating that adding TTSP can improve the surface stability of the materials during the cycling process. The electrochemical performance of the LLO cathodes was characterized using 2032 coin-type half cells, with a Li metal anode and different electrolytes. The cycling tests were performed between 2.0 V and 4.8 V at different current densities. One of the best performing cathodes with TTSPi delivered an initial discharge capacity more than 250 mAh g-1 at 0.1 C, with a capacity retention of 85% after 100 cycles, indicating largely improved electrochemical characteristics.
Moore-O3
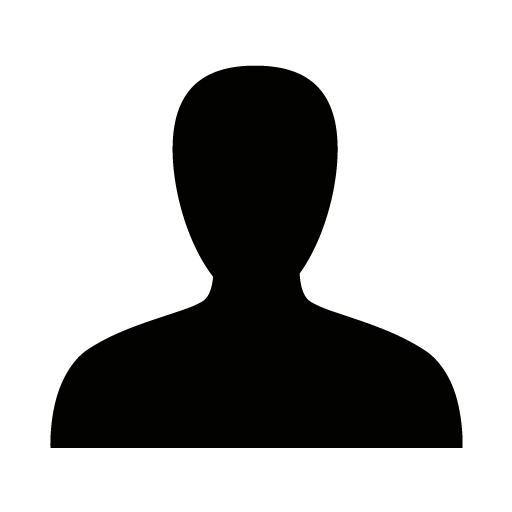
We developed a lithium ion battery intercalation & exfoliation method with detailed experimental procedures for the mass production of 11 two dimensional TMDs and inorganic nanosheets, such as MoS2, WS2, TiS2, TaS2, ZrS2, graphene, h-BN, NbSe2, WSe2, Sb2Se3 and Bi2Te3, among them 3 TMDs achieved mono- or double layer yield > 90%. This method involves the electrochemical intercalation of lithium ions into layered inorganic materials and a mild sonication process. The Li insertion can be monitored and finely controlled in the battery testing system, so that the galvanostatic discharge process is stopped at a proper Li content to avoid decomposition of the intercalated compounds. The intercalation strategy can also be used to tune 2D TMDs’ physical and chemical properties for various applications. For example, we developed an one-step covalent functionalization method on MoS2 nanosheets for membrane fabrication, which exhibited excellent water desalination performance. For lithium intercalation mechanism, the state-of-the-art In-Situ Liquid Phase TEM is an ideal technique for identifying the phase changes during intercalation process. With self-designed electrochemical liquid cell utilized, we can directly vapture the dynamic electrochemical lithiation and delithiation of electrode in a commercial LiPF6/EC/DEC electrolyte, such as LiF nanocrystal formation, lithium metal dendritic growth, electrolyte decomposition, and solid-electrolyte interface (SEI) formation. Combining with other in-situ techniques, such as in-situ XAS, XRD and Raman, etc, the underlying lithium intercalation mechanism in TMDs were further investigated, which render us a comprehensive understanding of the intrinsic correlation between the intercalation process and TMDs layered structures.
Moore-O1
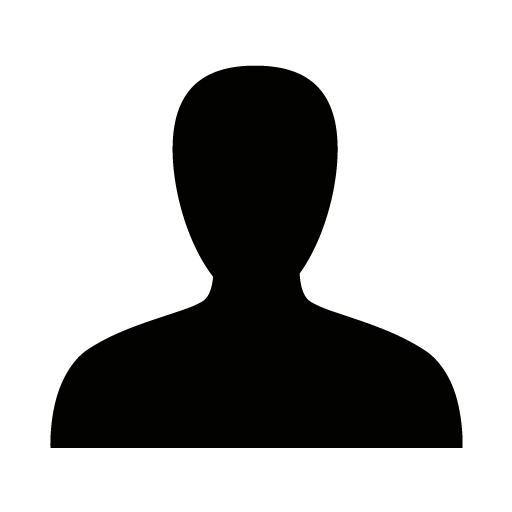
The chemo-mechanical instabilities in the cathode materials play a crucial role in the electrochemical performance of the alkali metal ion batteries. During battery operation, alkali metals (Li, Na, and K) intercalate into the cathode structure, leading to a fundamental shift in the electronic and structural properties of the cathodes. Intercalation also leads to continuous volumetric changes in the cathodes, which leads to severe mechanical deformations and, eventually, particle fracture. Furthermore, the interaction between organic electrolytes and cathode materials under an electric field could lead to the formation of cathode-electrolyte interphase (CEI) formation. Operating the batteries at higher voltages is desirable for high energy density operations; however, it further escalates structural and interfacial instabilities on the cathodes, resulting in faster capacity decay and shortened lifetime of the batteries. Charging the batteries at faster rates is also needed for demanding applications such as electric vehicles, but diffusion-induced stress and associated metastable phase formations in the cathodes could also lead to severe capacity loss in the batteries. Understanding the governing factors behind these interfacial and structural instabilities is crucial to designing robust battery materials. In this aspect, operando and non-destructive measurements are required to shed light on these instability mechanisms.
Here, I will start the talk by describing the principles of curvature measurement and digital image correlation (DIC) techniques for measuring stress and strains in the cathodes during battery operation. Both are optical and non-destructive techniques [1]. Curvature measurements provide information about stress generation in the electrodes with temporal resolution as a result of structural deformations and surface pressure build-up on the cathodes. DIC provides information about strain generations in the electrodes, with spatial and temporal resolution, as a result of volumetric changes in the composite electrodes during intercalation. In this talk, I will present specific examples of how these operando techniques shed light on interfacial and structural instabilities in cathodes, such as amorphization during intercalation, the formation of CEI layers, and capacity loss at high voltages. First, in-operando DIC measurements indicated that strain rates, rather than absolute strains, cause amorphization in the crystalline cathodes during ion intercalation [2]. Second, the operando DIC, coupled with cryo X-ray photoelectron spectroscopy, determined the onset of the CEI formation on the cathode surface in organic electrolytes. [3] Furthermore, the role of the transition metals on the electrochemical performance of cathode structures and associated chemo-mechanical instabilities were investigated by combining electrochemical analysis, DIC, and spectroscopy techniques. [4] The synchronization of in-operando curvature and DIC measurements also provided information about the governing forces behind the structural and interfacial instabilities in cathode electrodes at higher voltages. Overall, utilizing DIC and curvature measurements is a powerful tool to unravel charge storage mechanisms and governing forces behind interfacial and structural instabilities in electrode materials.
Moore-O2
The growth of lithium dendrites in inorganic solid electrolytes is an essential drawback that hinders the development of reliable all-solid-state lithium metal batteries. Generally, post mortem analysis of batteries show that grain boundaries in inorganic solid electrolytes are the preferential sites for lithium dendrite penetration. Operando Kelvin Probe Force Microscopy (KPFM) measurements elucidate the initial aspects of dendritic growth in a Li6.25Al0.25La3Zr2O12 garnet-type solid electrolyte. Strictly speaking solid electrolytes are mixed ionic-electronic conductors. Here KPFM maps the internal electrostatic (Galvani) potential change during battery operation. We find that the Galvani potential drops at grain boundaries near the lithium metal electrode during plating as a response to the accumulation of electrons [1]. Based on these results, we propose a mechanistic model to explain the preferential growth of lithium dendrites and their penetration in inorganic solid electrolytes [1]. In addition, the operando KPFM method enables the characterization of potential distribution across thin-film all-solid-state batteries. Thereby, we detect and follow the space charge layer evolution in a model Li|Li3PO4|LiCoO2 thin film battery at different states of charge in the voltage range from 3.0 V to 4.3 V vs Li+/Li. We find that a space charge layer mainly exists at the LPO|LCO interface. The contribution of the space charge layer to the whole interfacial resistance is >30% at voltages >3.9 V and reaches a maximum value of about 39 Ω cm2 at 4.3 V vs Li/Li+. Both examples of KPFM illustrate that the method is versatile and benefitial for characterizing and understanding batteries and their components [1].
Abbey-K1
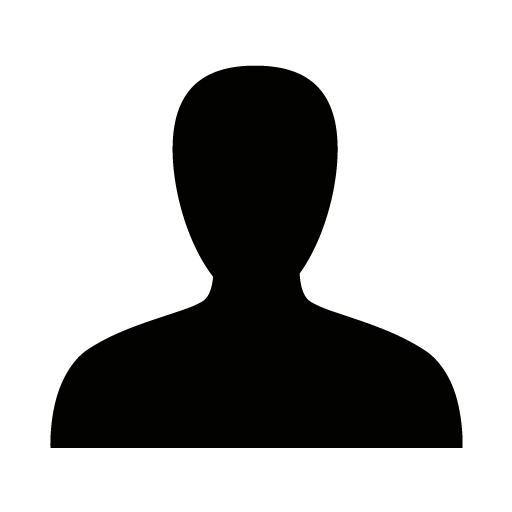
The surface layer, only one molecule thick, in reduced ceria has, in recent year, been the subject of experimental investigation applying XPS. Two compositions were examined (SDC) [1] and (PCO) [2]. In SDC the surface is highly reduced and the concentration of Ce3+ ions, [Ce3+], reaches about 30 cation%. In PCO, reduction is done at a higher oxygen pressure, P(O2), under which Pr4+ is reduced to Pr3+ but Ce4+ stays intact. The concentration of Pr4+ ions, [Pr3+], reaches at most 10 cation%. Theoretical analysis of the experimental [Ce3+] - P(O2) relations in SDC [3] and those of [Pr3+] – P(O2) [4] require that the surface of SDC becomes metallic while the bulks stays semiconducting. On the other hand, the surface of PCO is semiconducting. The latter difference between SDC and PCO surfaces is due to the difference in the charged defect concentration. The electrons on single Ce3+ ions and single Pr3+ ions are small polarons, i.e. localized and can propagate to nearby ions by hopping. However, under high defect concentration, as is the case in SDC, the electrons become delocalized and the surface becomes metallic. This is reminiscent of the Mott transition where in the bulk localized electrons become delocalized when their concentration exceeds a critical value.
The theoretical interpretation of the SDC experimental results shows that the surface is a two-dimensional metallic layer, one molecule thick on top of a poorly conducting semiconductor bulk. The interpretation provides the density of state of the metallic conduction band and the electron effective mass.
In PCO the theoretical interpretation yields that the defect concentration, [Pr3+], in the semiconducting surface is different from that in bulk and a double layer between the surface and bulk exists with the surface being negatively charged. This is in contrast to the case of SDC where there is no (or negligible) charge transfer between the metallic surface and the bulk and the surface is neutral.
The phase diagram (which reflects the ion and oxygen vacancy arrangement) of the metallic surface in SDC is examined based on the assumption that the ion arrangement in surface is forced to comply with the arrangement of ions in the bulk, as is the case in epitaxial growth. This forces the surface to maintain the a phase of the reduced SDC bulk [3] while a difference is allowed in the concentration of Ce3+ and Sm3+ between the surface and the bulk.
In the metallic surface of SDC the concentration [Ce3+] and hence that of oxygen vacancies is higher than in the bulk for a given temperature and P(O2). Similarly, in the semiconducting surface of PCO, the concentration [Pr3+] and that of oxygen vacancies is higher than in the bulk.
Abbey-O1
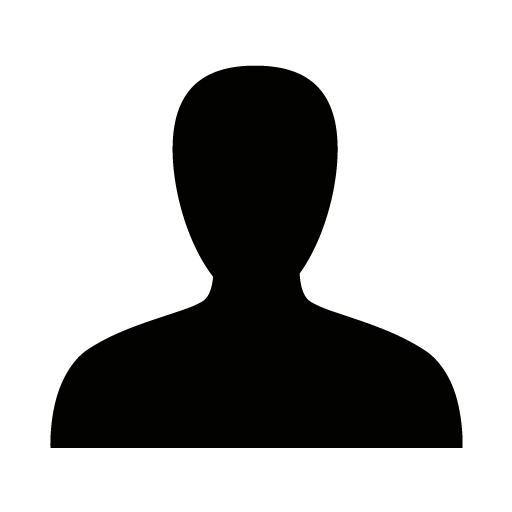
Protonic ceramics has attracted much attention as the potential material for electrochemical devices that reduce an environmental impact. Physical properties of these materials are restricted by the formation defect derived dopant and the conductivity is in proportion to the product of the proton concentration and diffusivity. Proton concentration depends on the amount of the oxide ion vacancy, which is formed due to charge compensation with acceptor dopants. Proton diffusivity depends on crystal structure and dopant arrangement. To achieve both a high proton concentration and fast proton diffusivity, we demonstrate fast proton conduction in nonstoichiometric barium zirconate, which could form a large number of defects without adding dopant. Nonstoichiometry of cation and oxide ion possibly incorporate proton into crystal and proton migration might be not limited by trap between proton and dopant. We tried to form the nonstoichiometric defect and enhance proton conduction by the exsolution technique used to disperse catalyst. To introduce a nonstoichiometric defect, lutetium- and nickel-codoped barium zirconate was reduced under hydrogen atmosphere and, then, nickel was exsolved. The exsolved sample shows the proton conduction, which exceeds 0.01Scm-1 in the temperature range of 400-800 ℃. We attempted to generate electricity in a fuel cell using this material as an anodic catalyst. The catalyst showed superior power generation properties for fuel cells at 500-650°C.
Abbey-O2
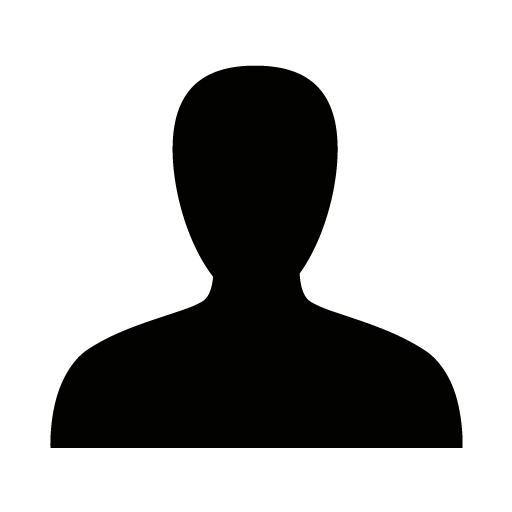
Catalysts play a crucial role in advancing energy conversion and storage systems, particularly in chemical and electrochemical reactions. A recent advance in this field has been the synthesis of self-assembled metal nanoparticles through a process known as “exsolution.” In exsolution, metal nanoparticles grow in situ, anchored to a host oxide, with perovskite oxides commonly serving as the parent oxide. Perovskites are important because they provide electron and ion conductivity, essential in energy-related applications such as fuel cells and electrolysis cells for power generation and hydrogen production.[1] Previous studies have revealed how external stressors can be used to tune the perovskite defects,[2] particularly oxygen vacancies at the surface, which will be key in determining the transport properties and the kinetics of exsolution.[1,2] A previous work from our group [2] showed that the density of exsolved Fe^0 nanoparticles from La0.6Sr0.4FeO3 (LSF) oxide matched the concentration of oxygen vacancies, revealing that oxygen vacancies are preferential nucleation sites for exsolution. Another study by Wang et al. from our group [3] showed how the Fe^0 exsolution also changed the bulk structural properties, creating Fe-deficient percolating channels that assisted the increase in electronic conductivity. However, the role of surface chemistry in exsolution kinetics and nanoparticle formation is not yet fully understood.[1]
In this work, we explored how the surface chemistry of La0.5Sr0.5Ti0.94Ni0.06O3 (LSTN) affects the exsolution kinetics of metallic nickel (Ni) nanoparticles. To investigate this, we modified the surface using various metal oxides through pulsed laser deposition. Previous work by Nicollet et al. [4] demonstrated that the acidity of binary oxides can influence oxygen surface kinetics in mixed conducting oxides. Building on this insight, we selected three metal oxides (MgO, ZnO, and SnO2) with varying acidity levels, as suggested by Nicollet et al. We hypothesize that the acidity of the metal oxide influences the exsolved nanoparticles’ surface area, particle size, and kinetics of Ni nanoparticles by affecting the formation of oxygen vacancies. The “surface decorations” adjust the concentration of oxygen vacancies, hence influencing the exsolution sites and properties of the nanoparticles. We assessed how adding different monolayers of binary oxides to LSTN thin films impacts the thermal exsolution of nanoparticles by using ambient-pressure spectroscopic techniques such as X-ray photoelectron spectroscopy and X-ray absorption spectroscopy.[1] Establishing correlations between surface chemistry, particularly acidity, and nanoparticle properties is crucial for controlling exsolution surfaces tailored for specific electrochemical reactions, such as hydrogen production through water splitting or carbon dioxide reduction.
Abbey-O3
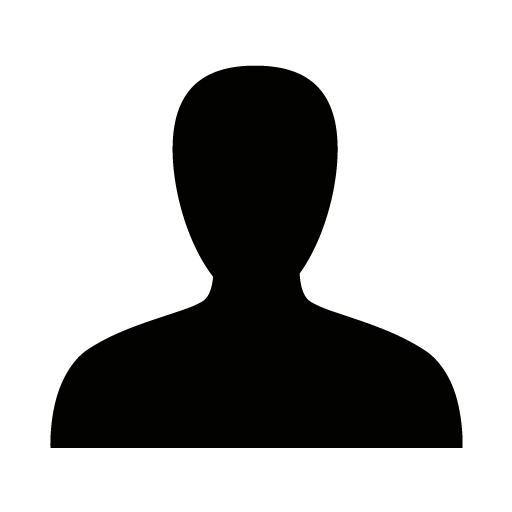
In our previous work, the CeO2 nanoparticles (NPs) decorated La0.43Ca0.37Ti0.94Ni0.06O3-δ (denoted as LCTN-Ce) electrode showed an excellent electrochemical performance of CO2 electrolysis with solid oxide cells (SOCs).[1] Herein, the effect of surface decorated CeO2 amount on the reversible SOC (RSOC) performance has been investigated in 50% CO2/50% H2 at 750 °C. Results show that with the increase of CeO2 amount, the single cell assembled with LCTN-Ce electrode (denoted as LCTN-Ce cell) exhibits a significantly enhanced CO2 electrolysis performance, owing to the increased oxygen vacancy concentration. While the output performance of LCTN-Ce cell increases first and then decreases, which is attributed to the reduced electrode conductivity. The maximum power density is measured to be ~350 mW cm-2 over LCTN-Ce005M-4h cell. Furthermore, this cell displayed a short-term stability for 12 h under the reversible operation of SOFC mode with a constant current load of 125 mA cm-2 and SOEC mode with a constant current load of 360 mA cm-2.
Abbey-O4
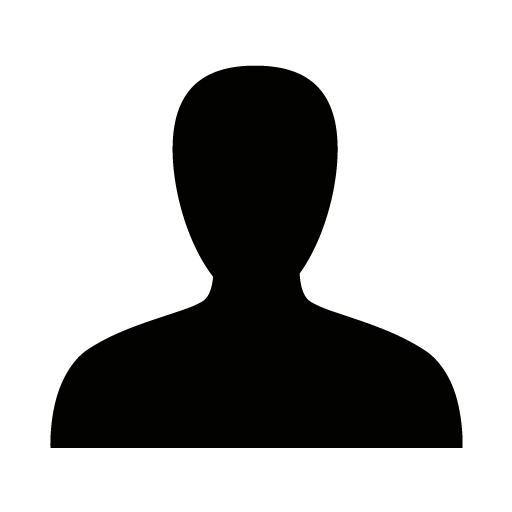
Current Solid Oxide cells (SOC) shows limited stability and lifetime considering the high working temperature 800-1000°C. One solution is to lower the working temperature allowing a larger range of usable material and better stability. However, reaction kinetics at the electrodes are sluggish at lower temperatures, which limits the cell performance. Then, one critical goal is to better understand the reaction pathways controlling the electrode kinetics, to be able to improve them. In the literature [1], it was shown for oxygen electrode that reaction kinetics at the electrode surface is directly dependent to the electrons availability at the surface, as well as the oxide ion conductivity of the electrode materials.
It was deduced that conduction properties of the electrode material had a direct impact on reaction kinetics and could be limited by oxygen reduction and O2- diffusion through the bulk. It was also shown that surface chemistry has a dramatic influence on oxygen reduction. Indeed, impurities at the surface of a mixed conductor can modify reaction kinetics by many orders of magnitude. In previous works, it was demonstrated that the effect of impurities on oxygen surface exchange kinetics scales with their acidity, determined with the Smith acidity scale [2,3]. Such effect has been shown to hold for various mixed conducting oxides used as oxygen electrodes [4,5], but there is no evidence that it can be applied to other catalyzed reaction, such as the H2O/H2 reaction at the fuel electrode.
In this work, we studied the influence of acidic/basic oxide impurities on reaction kinetics of fuel electrodes, and whether the trends observed on air electrodes can be extended to other gas-oxides reactions. The selected anode material is Gd0.10Ce0.90O1.95 as it has been proven to be an efficient standalone anode material [6]. Gd0.10Ce0.90O1.95 electrodes were prepared by screen‑printing on YSZ electrolytes, and the reactions kinetics were measured by impedance spectroscopy. Then, impurities were added in-situ by infiltration of nitrate solution followed by a calcination prior to impedance measurements. The variation of reaction kinetics are discussed with respect to the Smith acidity of the impurities, their loading, and their influence on the electronic structure of the surfaces of Gd0.10Ce0.90O1.95 anodes. Then, a more general discussion is proposed to compare those results with those obtained previously on cathode materials.
Abbey-O5
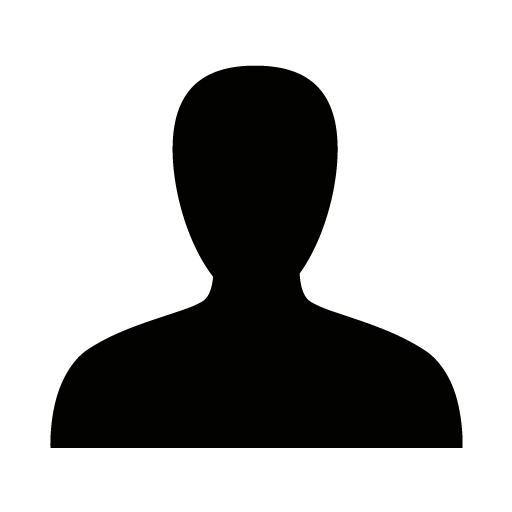
Oxygen transport through the best mixed ionic−electronic conducting oxides is often governed by the transfer of oxygen across the material’s surfaces rather than oxygen diffusion through the bulk phase. This transfer process is rather complex, involving oxygen containing molecules becoming oxide ions (or vice versa) in a multistep reaction with various reaction intermediates. Generally, the surface is simply taken as the planar interface between the gas phase and the solid. This simplification is problematic because it ignores that the surface has its own structure, which depends on the surface orientation, and that the surface may be charged, with an attendant space-charge zone within which charge-carrier concentrations are drastically altered.
Taking the prototypical perovskite SrTiO3 as a model system, we examined both the influence of the surface orientation and of the presence of water in the gas phase on the surface exchange coefficient and the surface space-charge potential was investigated [1, 2]. We employed two different isotope exchange experiments to investigate these parameters: either a dry (18O2 / 16O2) or a wet (H218O / H216O) isotope exchange followed by determination of the isotope diffusion profile with Time-of-Flight Secondary Ion Mass Spectrometry (ToF-SIMS). We obtained data for single-crystal samples of SrTiO3 with three different surface orientations, (100), (110) and (111), and for dry and wet atmospheres for the (100) surface orientation. Surprisingly, the difference in isothermal surface exchange coefficients measured for the different surface orientations was less than an order of magnitude. A further surprise was that the (110) and (111) surfaces exhibited a clear change in activation enthalpy, whereas the (100) surface did not. A third surprise was that the behaviour of the surface exchange coefficients was similar to that for the surface space-charge.
For two of these surprising results (the latter two), we found a single explanation. We started with a careful analysis of the surface exchange data, attributing the high activation enthalpy at high temperatures to a mechanism limited by charge transfer and the low activation enthalpy at lower temperatures to a mechanism involving adsorbed water species (due to trace amounts of water present in the oxygen exchange gas), which was further explained by the wet exchange experiments. The common behaviour of the two parameters is then linked to the presence of charged OH surface species at lower temperatures.
Gielgud-K1
Low-temperature growth with mild annealing to induce crystallinity is emerging as a route to exceptional catalytic activity in mixed ionic/electronic conducting oxides (MIECs) within a limited thermal budget. While our prior work focused on the key role of electronic conductivity enhancements during crystallization and the pristine surface chemistry in promoting surface charge transfer, little is known about the ionic conductivity of amorphous MIECs nor about its evolution during crystallization. In this work we developed a thin-film cell architecture involving blocking electrodes and capping layers to quantify ionic and electronic conductivities plus ionic transference numbers at each stage of crystallization, both isothermally and during thermal excursions. Using a (La,Sr)(Ga,Fe)O3-x perovskite as a model system, we observed a ~2 orders of magnitude increase in ionic conductivity during crystallization. However, the total conductivity increased ~4 orders of magnitude. As a result, the material transitioned from being nearly a pure ionic conductor to nearly pure electronic conductor as crystallinity varied. Transference numbers over almost the full range from ~0 to ~1 could be dialed in without changing the nominal composition. By combining ac-impedance spectroscopy with simultaneous synchrotron grazing-incidence X-ray diffraction, dc polarization studies, TEM imaging, optical properties, and molecular dynamics simulations, we were able to evaluate the relative roles of lattice order and strain, defect chemistry, and microstructure in the evolving transport behavior with crystallinity.
Gielgud-O1
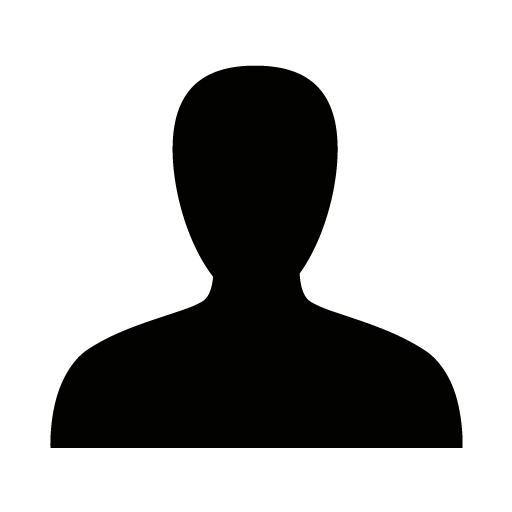
Novel high- and medium-entropy SrFe1-xMoxO3-δ-based transition metal-doped perovskites are synthesized for the first time, and assessed as potential electrode materials for both conventional and symmetrical Solid Oxide Fuel Cells (SOFC). Four compositions: SrFe0.8Mo0.2O3-δ (SFM), SrFe0.6Mo0.2Co0.2O3-δ (SFMC), SrFe0.4Mo0.2Co0.2Mn0.2O3-δ (SFMCM), and SrFe0.2Mo0.2Co0.2Mn0.2Ti0.2O3-δ (SFMCMT) are obtained by a solid-state synthesis method. For all materials, the structural analysis, with the use of XRD and SEM measurements, confirms presence of the single-phase structure. The oxygen nonstoichiometry level determination shows very interesting behavior, which is especially visible for the high-entropy SFMCMT composition, strongly deviating for prediction of the rule-of-mixtures. To assess the potential of those materials regarding symmetric-type operation (both as cathode and anode), their structural evolution under reducing conditions is studied, indicating a degree of either exsolution or decomposition for all compositions. In the first case, such behavior should, at least in theory, enhance the catalytic properties of the materials under a reducing atmosphere. Most importantly, the analysis of the SFMCMT sample upon further reoxidation indicates at least partially reversible exsolution of the catalytically active Co- and Fe-rich metallic precipitates. The confirmed reversibility is a highly beneficial feature in terms of application in symmetrical SOFCs, indicating that the single-phase structure can be restored after reversing the polarization direction of the operating cell. The functional properties of all materials, including electrical conductivity, thermomechanical properties, and chemical stability toward the most common solid electrolyte materials, is studied. The selection of the B-site elements appears to have a prominent impact on the transport properties, in terms of the conductivity values, and the general character of its temperature dependence. The thermomechanical measurements indicate that the high-entropy approach leads to a significant decrease in the thermal expansion coefficient, with the recorded TEC for the SFMCMT equal to 13.1·10-6 K-1 and 15.1·10-6 K-1, below and above 500 °C, respectively. Such behavior is highly desired from the point of view of compatibility with other elements of the fuel cell, as the obtained values are closely matching the ones of typical electrolytes. Consequently, the SFMCMT is selected for further evaluation of the electrochemical performance, under both oxidizing and reducing conditions. The electrochemical impedance spectroscopy measurements prove that cathodic polarization resistance values for SFMCMT material are significantly lower than those for the conventional SFM material, obtained in the same experimental conditions. Overall, the high-entropy SFMCMT perovskite demonstrates a significant improvement in performance over the legacy SFM composition, making it a highly promising alternative for application in conventional and symmetrical SOFCs.
Gielgud-O2
Compositionally complex oxides (CCOs) are an emerging material class that includes high-entropy oxide (HEOs) and entropy-stabilized oxides (ESOs), whose unprecedented properties stem from disorder-induced distributions in electronic structure and chemistry caused by stabilizing many-cation (typically > 5) solid solutions [1,2]. This contribution presents our lab’s recent works elucidating the formation mechanisms and property implications of CCO-derived composite and thin film electroceramics, with a theme of atomic- and nanoscale characterization by scanning transmission electron microscopy (STEM).
Research on HEOs/ESOs has primarily focused on exploring new structures, chemistries, dislocations [3], or unique properties. However, few studies discuss the impact of secondary phases on functionality. Here, electrical transport mechanisms in the canonical ESO (Co,Cu,Mg,Ni,Zn)O were assessed as a function of secondary phase content [4]. When single-phase, the oxide is a small polaron electronic conductor. After heat treatments, Cu-rich tenorite particles form at grain boundaries, which enhances the grain interior rocksalt oxide electronic conductivity due to increased Cu cation vacancies and compensating small hole polarons. While Cu depletion tailors grain interior the conduction mechanism, Cu-rich tenorite grain boundary phases create a pathway for Cu2+/Cu3+ small hole polarons after longer heat treatment times. The ability to selectively grow secondary phases nucleated at grain boundaries enables tuning of electrical properties in CCOs, HEOs, and ESOs using microstructure design, nanoscale engineering, and heat treatment, paving the way to develop many novel materials.
Integrating novel CCOs/HEOs/ESOs into composites with nanoscale tunability, using methods such as self-assembly [5] and exsolution [6], will enable tailored (multi)functionality beyond what is possible in a single phase. Here, we demonstrate a novel, highly extensible approach, exsolution self-assembly (ESA), to realize CCO-based nanocomposite thin films with intricate multi-element nanostructures [7,8]. Using pulsed-laser deposition (PLD) [9], we selectively reduce cations in a model perovskite CCO LaFe0.7Ni0.1Co0.1Pd0.05Ru0.05O3-δ, inducing defect-interaction-driven exsolution and simultaneous self-assembly of metal nanorods and metal-oxide core-shell nanoparticles, depending on oxygen partial pressure (PO2). A correlated analysis using STEM imaging and spectroscopy, strain mapping, atom probe tomography with 3D mass spectrometry, and X-ray photoemission spectroscopy was performed to characterize the ESA nanostructures and elucidate the nanostructure formation mechanisms underlying the highly tailorable synthesis approach. With decreasing PO2 from 3 mtorr, 0.15 mtorr, to 0.015 mtorr, concentration of oxygen vacancy increases, which tunes the extent of exsolution for different ESA nanostructures. At PO2 of 3 mtorr, the LaFeO3-based CCO thin film matrix shows uniform cation distribution. When PO2 drops to 0.15 mtorr, ESA Pd nanorods grow from bottom of the thin film to top surface, with growth restricted by compressive stress exerted by the matrix in the in-plane direction and Pd availability in surroundings. When PO2 further reduce by 10 times to 0.015 mtorr, Pd-NixCo1-xO metal-oxide core-shell nanoparticles embedded in the matrix form via seed growth effect triggered by growth of Pd followed by subsequent exsolution of Ni3+ and Co3+ in the CCO matrix. ESA is expected to synthesize complex and multi-dimensional nanostructures for electrochemical devices via integration of novel compositions and crystal structures of CCOs as well as PLD conditions.
Gielgud-O3
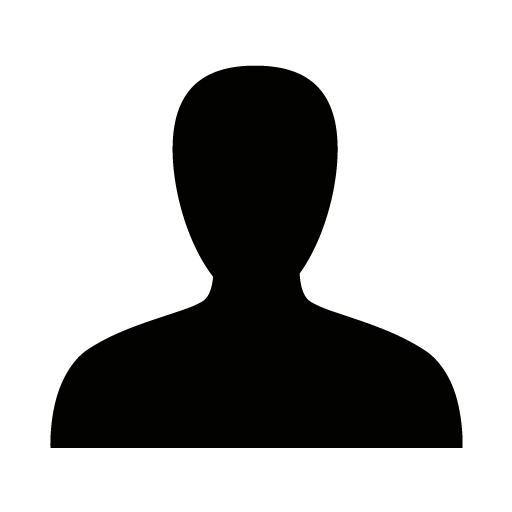
While solid oxide cells (SOCs) offer a promising technology for energy production and storage, their extensive commercialization faces challenges, primarily associated with high degradation rates. Although both fuel and oxygen electrodes are susceptible to distinct degradation mechanisms, substantial efforts have been dedicated to the comprehension of the underlying factors contributing to the diminished performance of the air electrodes. A proposed strategy to mitigate the degradation of these electrodes and increase their stability involves increasing the entropy of the material [1,2]. This research seeks to tackle the air electrode degradation challenge by investigating a perovskite material composed of high entropy oxide (HEO).
The material of choice in this work is a HEO material which in the A-site of the perovskite structure contains several lanthanide group elements and with various transition metals (TM) occupying the B-site thus increasing the configurational entropy from 1.17R for LSCF to 2.94R for our HEO. The synthesis of this HEO material was accomplished using the Pechini method, a widely employed technique known for its effectiveness in producing finely powdered materials [3,4]. In this work, using the Pechini synthesis method, a single-phase perovskite structure of the high entropy oxide was obtained.
The synthesized HEO powder was then incorporated into a commercial anode-supported cell for comprehensive evaluation in both solid oxide fuel cell (SOFC) and solid oxide electrolysis cell (SOEC) environments. The ensuing results revealed a remarkable achievement – at 900 oC, a current density of 1.75 A·cm-2 and 0.7 V was achieved. This performance stands out as one of the highest recorded current densities for a cell utilizing HEO materials.
Beyond the great performance, a key aspect of this HEO's performance lies in its exceptional stability over an extended period. Even when subjected to high current densities, such as 1 A·cm-2, the material demonstrated remarkable stability, maintaining consistent behavior for more than 500 hours. This extended operational durability is a critical factor in advancing the practical application of HEO materials in solid oxide fuel cells, addressing one of the key challenges faced by the technology.
In conclusion, the integration of HEO as air electrode into the SOC framework represents a significant leap forward in addressing the challenges hindering the widespread commercialization of solid oxide fuel cells. The high current density and prolonged stability observed in this research underscore the potential of HEO materials to redefine the landscape of SOC technology.
Gielgud-O4
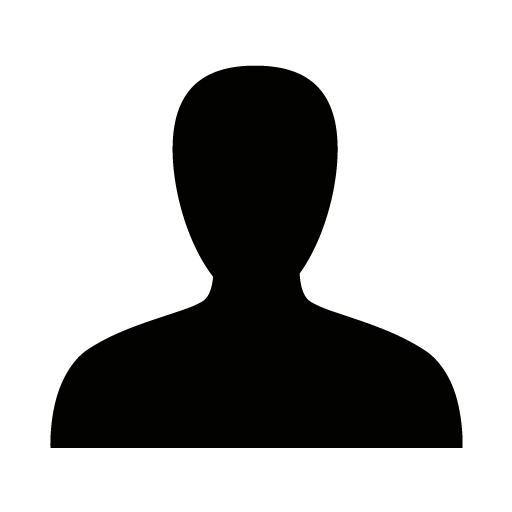
Since the goal of net zero emissions has been set, a great attention was directed towards the novel environmental-friendly energy technologies. Hydrogen appears as the promising option due to its carbon-free emission [1]. Solid Oxide Cells (SOCs), known as a clean energy technology, could be operated in either fuel cell or electrolyser mode, which is crucial to the development of energy technologies in modern society [2]. Meanwhile, it was shown that the oxygen electrode often limits SOC performance, derived from its large polarization resistance at intermediate temperature (600-800 °C). Thus, studying and developing novel oxygen electrode materials is indispensable, as it should maintain desirable performance output for the prolonged operation time [3].
Currently, high-entropy oxides (HEOs) have appeared as a novel option in materials science, due to their high configurational entropy, generated by introduction of diverse elements at either a varied or equimolar proportion. HEOs may exhibit enhanced physicochemical properties, compared to the typical perovskite-type oxides that are applied as electrode materials in SOCs [4]. Meanwhile, the cobalt-containing perovskites are still widely-used as oxygen electrodes in SOCs, ascribed to their remarkable electrocatalytic activity. Nevertheless, environmental toxicity and high capital costs of cobalt should be highlighted. Therefore, based on the adjustable characteristics of HEOs, and the similarity of other 3d metal elements (e.g. Mn, Fe, Ni and Cu), novel candidate oxides based on single perovskite skeleton can be proposed, curbing the negative effects of the cobalt element and maintaining the favorable catalytic activity at working temperatures.
In this work, multicomponent La0.6Sr0.4Ni0.15Mn0.15Fe0.15CuxCo0.55-xO3-δ (LSNMFCC) perovskite-type oxides were studied as oxygen electrode materials for SOCs. A role of copper substitution for cobalt and the influence of the increased entropy were evaluated. Electrospinning was adopted for morphological modification. Characterization methods include X-ray diffraction measurements at different temperatures, iodometric titration, scanning electron microscopy, pseudo 4-probe electrical conductivity and Seebeck effect tests, to assess lattice structure during heating/cooling processes, oxygen nonstoichiometry, microstructural and charge transport characteristics, respectively. For the electrocatalytic activity and performance, symmetrical and full cells were prepared with selected electrodes. La0.8Sr0.2Ga0.8Mg0.2O3-δ (LSGM) and Ce0.9Gd0.1O2 (GDC) were used as electrolyte in symmetrical cells, while LSGM was selected for electrolyte-supported full cells.
The as-synthesized LSNMFCC materials, by either sol-gel or electrospinning method, all crystalize in the R-3c symmetry, the structure is stable up to 900 °C. Specifically, no impurities or inhomogeneity could be detected among compositions with x=0.05, 0.1, 0.15 and 0.2. Selected four oxides exhibit similar oxygen nonstoichiometry at room temperature (δ ≈ 0.12-0.14). The total conductivity of the materials is very high in the range of 25-850 °C, above 200 S cm-1. The negative Seebeck coefficient indicated the electrons is the charge carriers. Symmetrical cells with LSNMFCC electrodes were prepared and tested. The studies revealed the lowest polarization resistance at 850 °C is 0.015 Ω cm2 for the x = 0.05 (LSNMFCC0550) electrode obtained by sol-gel method, while a little bit higher value of 0.017 Ω cm2 obtained for x = 0.2 (LSNMFCC2035) electrode at 850 °C. Since LSNMFCC2035 has lower cobalt content and exhibits higher entropy, it was selected for further modifications. After electrospinning, the polarization resistance of LSNMFCC2035 electrode could be decreased to 0.014 Ω cm2 at 850 °C. In further tests with full cell configuration, a maximum power density over 1.1 W cm-2 at 850 °C could be reached, with promising performance obtained also in the electrolysis mode. Those results imply that the optimized LSNMFCC can be applied as the perspective oxygen electrode material in SOCs.
Gielgud-O5
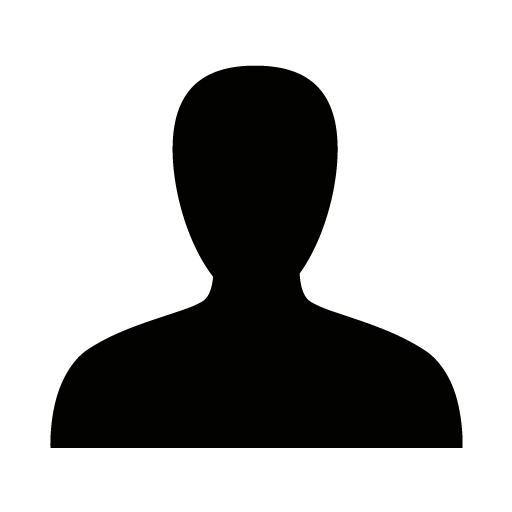
The fluorite structure is an extremely flexible structure, as it permits both anion vacancies and interstitials. This has had led to many applications in ionic conductivity and catalysis, and applies to a host of fluorites based on oxides, oxyfluorides, fluorides, hydrides, and oxyhydrides. We have previously reported on some lanthanide oxyhydrides based on the fluorite structure [1], and their ionic conductivities have been reported [2]. Separately, recently many high entropy oxides of the fluorite [3], bixbyite [3], and pyrochlore structure [4] have also been reported. Although these three structure types are related by their anion vacancy amounts, less characterization has been made on the precise anion content and coordination sites.
We will present our results on 2 high-entropy lanthanide oxides and oxyhydrides, (La0.2Ce0.2Pr0.2Nd0.2Y0.2)H1.5O0.75, (La0.2Ce0.2Pr0.2Nd0.2Y0.2)O1.5+𝛿. Based on a careful examination of X-ray and neutron diffraction data, we find that the high entropy effects of the lanthanide cations cause some interesting structural features not seen in the single-element analogues. For (La0.2Ce0.2Pr0.2Nd0.2Y0.2)H1.5O0.75, we see hydride exclusively occupying a certain interstitial site. The site location, and its selectivity is not reported in other known single-element LnH3-2xOx analogues. A low activation energy for hydride conductivity is observed, perhaps due to these interstitial sites. We also find that for corresponding high entropy distorted/oxygen-deficient fluorite LnO1.6, there are signs of local cation order between the 2 lanthanide sites based on size. Further studies on control over oxygen cotent, and its effect on structure, oxide ionic conductivity, and oxidation catalysis are underway.
Fleming-T1
Prof. Aron Walsh holds the Chair in Materials Design at Imperial College London. He received his PhD in Chemistry from Trinity College Dublin and later worked at the National Renewable Energy Laboratory, University College London, and the University of Bath. His research combines technique development and applications at the interface between solid-state chemistry and physics. He was awarded the EU-40 prize from the Materials Research Society for his work on the theory of solar energy materials, and is an Associate Editor for the Journal of the American Chemical Society.
The landscape of solid-state materials research is transforming with the integration of new techniques and tools from the artificial intelligence (AI) community. These changes are being facilitated by progress in hardware, including classical supercomputers and emerging commercial quantum computers, alongside software advancements incorporating advanced algorithms and statistical machine learning models [1]. Recent developments such as large language models and diffusion-based generative techniques are unlocking application areas ranging from teaching aides to self-driving laboratories. I will introduce the field of data-driven materials research, highlighting its potential to enhance chemical discovery and expedite the identification of compounds essential for advancing the next generation of clean energy technologies [2,3]. It will include insights from recent progress in understanding the nature of superionic, as well as mixed ionic-electronic conducting materials, while addressing obstacles such as reliable structure-property databases to enable more powerful models and the need for transparent reporting to ensure they are repeatable and reproducible [4].
Fleming-K1
This paper develops a model of a gadolinium-doped ceria (GDC) as a ceramic mixed ionic-electronic conducting (MIEC) electrolyte membrane in a membrane reactor. In applications such as fuel cells, the membrane is polarized via the chemical potentials associated with a fuel and oxidizer on opposite sides of the membrane. The membrane’s primary purpose is to conduct oxygen ions, with electrons being delivered to an external circuit. In fuel cells, because the electronic leakage through the membrane, which increases greatly above 600 ˚C, represents an efficiency loss, GDC-based cells are operated at relatively low temperature (T < 600 ˚C) where the electronic conductivity is relatively low.
The present application uses feed streams of H2O and CO that are separated by a GDC membrane and without any external electrical power. The purpose is the produce H2, via an electrochemically assisted process. The exothermic water-gas-shift reaction (H2O + CO = H2 + CO2, DH = 42 kJ/mol) provides the chemical potential needed to drive the membrane reactor. Unlike in fuel cells, electronic conduction (via a reduced-ceria small polaron) is an advantage for increasing charged-defect fluxes across the GDC membrane. This favors relatively high temperature operation.
The GDC membrane is sandwiched between porous composite cermet electrodes (e.g., Ni-YSZ). The oxygen conduction is represented as oxygen vacancies and the electronic conduction via reduced-ceria small polarons. The H2O reduction and CO oxidation are electrochemical charge-transfer processes that proceed at three-phase boundaries within the composite electrodes. Within the electrode structure, electrons are mobile in electrode phase (e.g., Ni), oxygen vacancies are mobile in the electrolyte phase (e.g., YSZ), and gases are in the pore phase. Nickel also serves as a heterogeneous catalyst.
The presentation develops the electrochemical and transport theory to model the process and uses computational simulation to predict performance. Some of the underpinning theory and model formulation were documented in the context of a GCD-based fuel-cell [1]
Fleming-O1
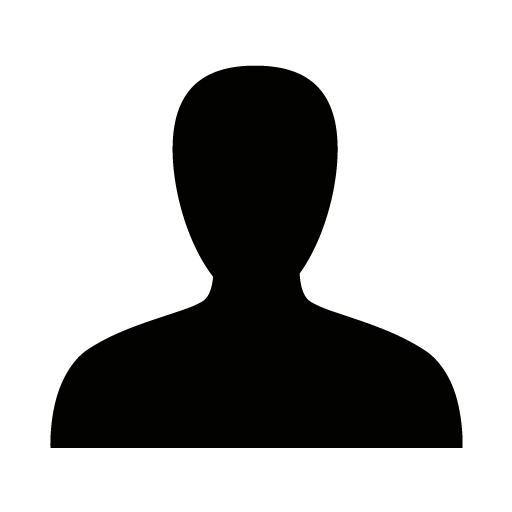
Methane (CH4) is a potent greenhouse gas. One potential solution to limit the emission of CH4 is to convert or upgrade it to value-added products. Oxidation of C-H bond in methane under mild conditions constitutes longstanding challenges which are of fundamental and technological importance, from biological and industrial to environmental. Earth’s atmosphere relies on free radicals to remove climate-damaging methane and other organic pollutants from the air. In recent decades, intensive efforts have been focused on leveraging free radicals for methane oxidation. Free radicals are one of key mediators underpinning many active sites in thermal catalysis and these enhancement effects induced by the light and electric fields. However, it remains unclear how free radicals as active species mediate the C-H bond activation in methane and dictate the products distribution.
In this talk, we will discuss our recent advances in photocatalytic oxidation of methane to methanol which combined rational design of photocatalysts, photocatalytic studies, as well as in-situ free radical detection. Our results unravel interactions between these free radicals and the solid co-catalysts, ions, and gases molecules in water, as well as their impact on methane removal and its selective oxidation. Our studies highlight the neglected while important interplay between photogenerated radicals and co-catalysts, ion impurities, and gas molecules in promoting methane removal and controlling the products distribution. Furthermore, we report an inherent trade-off in the photochemical methane to methanol conversion. A new design principle featuring isolating different reaction steps is developed to circumvent this trade-off.
Such molecular-level insights into photocatalysts and their interactions with free radicals are expected to promote the methane removal and its selective functionalization and offer informative design principles for chemical bonds activations that are driven by light, thermal catalysis, and electric fields.
Fleming-O2
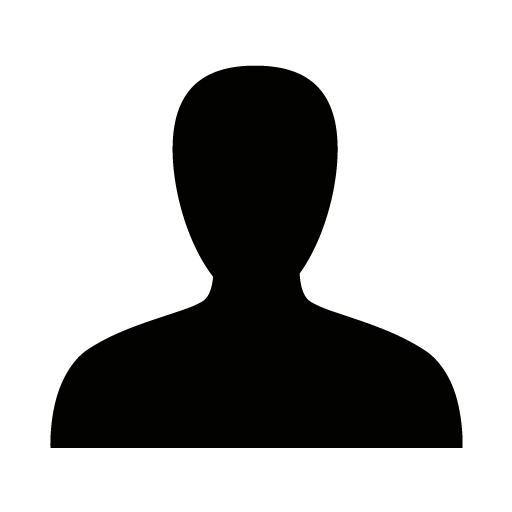
Direct air capture (DAC) has emerged as a pivotal technology for mitigating global CO2 emissions and achieving NetZero by 2050. Established DAC technologies typically employ alkanolamine or aqueous alkaline absorbents for CO2 capture, yet they incur high capital and operation costs1-3. These methods necessitate thermal cycling for CO2 capture and absorbent regeneration, exacerbating the process's carbon footprint. For example, thermal cycling process for alkaline absorbents alone incurs an energy cost of approximately 8 GJ/tCO21.
The electrochemical DAC (eDAC) system offers a distinct approach to CO2 capture and absorbent regeneration at a lower energy cost. Electrochemical capture methods commonly employ either organic or inorganic capture species4,5. While the inorganic strategy using alkali hydroxide absorbents enables stable atmospheric CO2 capture and release, it often demands a high energy cost (6–10 GJ/tCO2). Conversely, employing organic molecules has shown relatively lower energy consumption for electrochemical CO2 capture and release methods. However, the functional groups of such organic molecules are susceptible to oxidation by atmospheric oxygen (O2), particularly at DAC conditions where the O2 concentration exceeds that of CO2 by 500-fold.
Here, we present a hybrid approach that achieves stable and efficient atmospheric CO2 capture and release using alkali hydroxide absorbents (KOH) and organic redox mediators (Fig. 1, RM). This strategy combines the durability of alkali hydroxide capture absorbents with the fast kinetics of organic redox-active mediators. Employing a two-electrolyser electrocatalysis system, we operate the hydrogen evolution reaction (HER) in one electrolyser to generate OH- for CO2 capture, and the hydrogen oxidation reaction (HOR) in another electrolyser to generate H+ for CO2 release. The organic RM is oxidized and reduced at the counter electrode of the HER and HOR, respectively. With this approach, we achieve a minimum work of 0.82 GJ/tCO2, calculated based on the cyclic voltammetry redox potentials, and as low as 3.8 GJ/tCO2 in a practical two-electrolyser configuration with over 200 hours of stable operation.
Additionally, we introduce a new air contactor design that harnesses ambient wind to capture CO2 in the form of solid K2CO3 precipitates using a KOH solution and carbonate crystallizer. We demonstrate high-rate DAC capabilities of our carbonate crystallizer that are pertinent to large-scale industrial deployment, achieved without the need for costly equipment such as fans, packing, pumps, and the associated complex system designs. This approach yields a significant reduction in both capital and operation cost of the eDAC system.
Westminster-K1
Saiful Islam is Professor of Materials Science at the University of Oxford. He grew up in London and obtained his Chemistry degree and PhD from University College London. He then worked at the Eastman Kodak Labs, New York, and the Universities of Surrey and Bath.
His current research focuses on understanding atomistic and nano-scale processes in perovskite halides for solar cells, and in new materials for lithium batteries. Saiful has received several awards including the 2022 Royal Society Hughes Medal and 2020 American Chemical Society Award in Energy Chemistry. He presented the 2016 BBC Royal Institution Christmas Lectures on the theme of energy and is a Patron of Humanists UK.
Further breakthroughs in high energy density lithium-ion batteries require advances in new compositions and underpinning materials science. Indeed, a greater fundamental understanding into battery materials require atomic-scale characterisation of their ion transport, electronic and local structural behavior, which are important for optimizing performance. In this context, combined modelling-experimental work has been a powerful approach for investigating these properties at the atomic scale. This presentation will describe such studies in two principal areas: (i) investigating redox processes and nanostructures of Li-rich layered oxide and disordered rocksalt compounds as promising high capacity battery cathodes; here, the atomic-scale mechanisms governing redox behaviour in Li-rich structures are not fully understood; (ii) cation doping, defect clustering and ion transport mechanisms in solid electrolyte materials for solid-state batteries; here, we investigate two families of materials attracting growing interest as fast-ion conductors for battery electrolytes: anti-perovskite oxyhalides and ternary scandium halides. Microscopic effects are considered, and the results are used to rationalise macroscopic observations to guide future work on optimising battery performance.
Westminster-O1
Solid state battery (SSB) has attracted much attention as a promising successor to Li-ion battery (LIB). For full commercialization, however, there still remain crucial issues; finding super-ionic conductors, and control of the bottlenecks, which often appear around various types of interfaces, for ion transport as well as degradation. These interfaces, in fact, are affected by strain/stress owing to the intrinsic lattice mismatch around the interfaces and the volume change of electrode materials during charging/discharging. Though strain/stress effects have been examined on the meso- or macro-scopic scale so far, the atomistic studies were still limited. In this work, we explored the microscopic effects of strain/stress on the Li-ion transport by investigating (1) cathode materials (LixCoO2, x<1), and (2) cathode / coating layer interfaces (LiCoO2/LiNbO3, LiTaO3) by density functional theory (DFT) calculations.
First, we calculated Li-ion self-diffusion coefficients (D*Li)of LixCoO2,(x=0.81 and 0.69) under some strain/stress conditions via extensive DFT molecular dynamics (MD) simulations. We found that "Li layer distance" does not alter much upon the strain/stress conditions, and D*Li is more correlated with the “Co layer distance", which is in contrast to the chemical intuition. The detailed examinations demonstrated that the Co-Li electrostatic repulsion dominantly affects the Li-ion diffusion, and the Li-Li correlation also has effects. Besides, activation volume (Va) for cathode material was evaluated with the extension into the tensor form, which is theoretically for the first time. This extension can be utilized under different mechanical cases and anisotropic structures like a layered rock-salt structure.
For Li-ion migration across interface, we also investigated LiCoO2 interfaces with the coating materials LiNbO3 and LiTaO3, which have identical structures with slightly different lattice constants. Thus, the strain effects may be manifested in their comparison. Migration barriers across the interfaces by DFT calculations with Nudged Elastic Band method indicated that LiTaO3 has smaller barriers than LiNbO3. This tendency was consistent with the calculated Li chemical potential (minus of Li vacancy formation energy), in which the difference between LiCoO2 and the coating material is smaller in LiTaO3. Such difference can be attributed to the interfacial strain effect related to the different lattice mismatches in both coating materials. With more detailed analysis of the atomic structures during the migration, we extracted the characters correlated with the migration barriers.
In the talk, I will show more recent results and discuss the strain/stress effects from more general viewpoint, which will contribute to understanding and control of Li-ion transport in SSBs.
Westminster-O2
Dislocations have been observed in layered transition-metal oxide cathodes both in cycled and uncycled materials. They mediate stacking transitions which accompany phase transistions and also play a role for relaxing coherency strains. Since irreversible glide due to lattice invariance or local compositional changes can initiate a catastrophic sequence of degradation mechanisms a better understanding of the nature of dislocations is needed.
In this study we present explicit ab initio atomistic dislocation models of LiCoO2 and LiNiO2 by combining density functional theory and anisotropic linear elasticity theory. We characterize screw dislocations and find a peculiarly compliant behavior of LiNiO2 due to the interaction of Jahn–Teller distortions with the dislocation strain field. Moreover, we determine the segregation tendency of Li vacancies to dislocation core. Finally, we characterize stacking fault energies as a function of Li content and quantify the extent to which excess Ni hinders stacking-sequence changes. The results shed light on the role of dislocations for understanding and optimization of the chemomechanical behavior of cathode active materials during battery operation.
St.James-K1
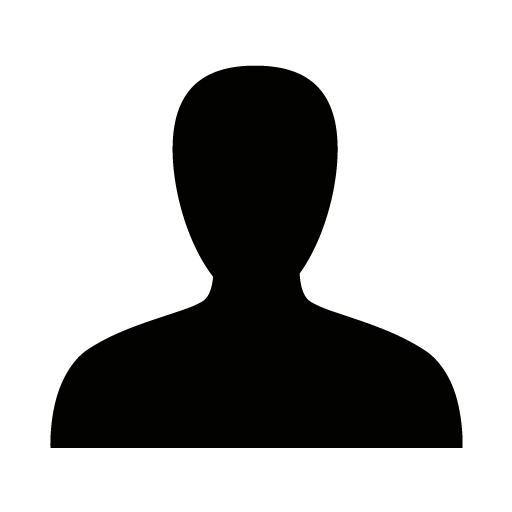
One of the expected advantages of alkali solid-state batteries is the potentiat utilization of metal anodes. This requires reversible, dendrite-free and long-term stable operation of either lithium or sodium metal anodes in contact with solid electrolyte separator layers. This lecture will summarize the fundamental key challenges for successful implementation of metal anodes and will present novel experimental results on the kinetics, the microstructure and morphology of lithium and sodium metal anodes in cells with a chemically stable oxide solid electrolyte. In particular, the pore formation under anodic load, as well as metal nucleation under cathodic load has been studied, along with the microstructure of the metal anodes. The microstructural evolution of the metal under kinetic load will be discussed in detail.
In addition, recent results on the silicon anode in sulfide based solid-state batteries will be presented [1]. It is demonstrated that planar and compact silicon layers may be well used as anode in lithium-based SSBs, once the mechanical strain exposed by the silicon anode is properly compensated. Composite anodes of silicon and a sulfide electrolyte suffer from severe SEI formation and may require more advanced interlayer concepts.
St.James-O1
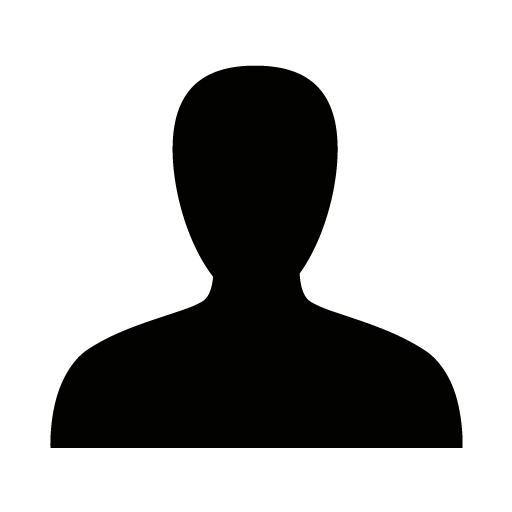
The field of sodium-ion batteries (SIBs) is rapidly gaining popularity, with SIB technology potentially offering a lower cost and more sustainable alternative to lithium-ion batteries (LIBs).[1], [2] Extensive research is being undertaken on the anode and cathode, however, the electrolyte for sodium-ion batteries is relatively underdeveloped. Sodium analogues of salts transferred from LIB technology, such as sodium hexafluorophosphate (NaPF6), have been studied and used commercially, however, there is a drive to develop novel electrolyte salts to improve battery performance, as well as focusing on sustainability and safety factors.
This work focuses on the development of a screening method for novel electrolytes, using model borate salts [NaB(OR)4] to understand the effect of solvent and binder choice on the cyclability of SIBs.[3] Pairing electrochemical impedance spectroscopy (EIS) with full cell galvanostatic cycling allows the SIB performance to be evaluated, as well as the solid-electrolyte interphase (SEI) stability to be investigated. In this study we show that a less stable interface in sodium-sodium symmetric cells is a good indicator of poorer overall cycling performance in a full cell. Sodium pinacolato-borate, Na[B(pp)2] is used with a variety of linear and cyclic carbonates; 1M Na[B(pp)2] shows poorest performance with propylene carbonate (PC), compared with co-solvent mixtures of ethylene carbonate (EC), with dimethyl carbonate (DMC) or diethyl carbonate (DEC) in a 1:1 volume ratio (EC:DMC and EC:DEC 1:1 v/v).
Compositional studies with nuclear magnetic resonance (NMR) and X-ray photoelectron spectroscopy (XPS) allow further insight into the formation and chemical composition of the SEI, and its effect on battery performance. The presence of sodium fluoride (NaF) is confirmed by both techniques as a key SEI component with these electrolytes, however, in lower amounts relative to the bulk anion, compared with NaPF6.[4] The choice of binder used in anode construction is also shown to have an effect on the composition, with polyvinylidene fluoride (PVDF) and carboxymethyl cellulose (CMC) reacting differently with degradation products inside the battery to give different amounts of NaF.[5]
St.James-O2
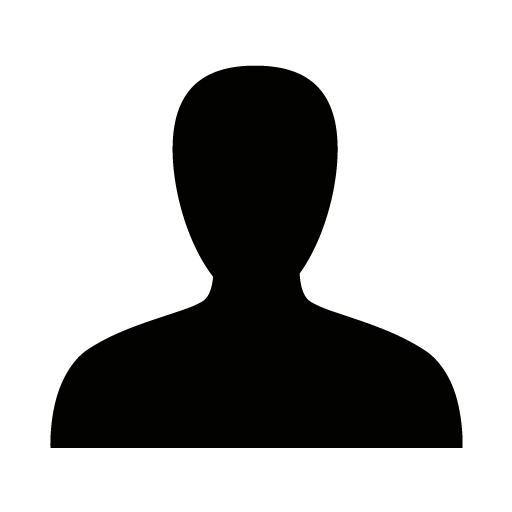
Na-ion battery (NIB) research is blooming at a rapid scale with the main purpose of reducing our extensive dependence on Li-ion batteries to fulfill the ever-increasing energy demands. While the Na-ion battery is effectively used for stationary applications, only the development of new high power and energy density cathode materials will increase the horizon of their utility. Layered transition-metal oxides (TMOs) are the state-of-the-art Na-ion battery cathode frameworks. However, the structural instability of TMOs at their fully desodiated state and detrimental phase transitions have directed the research towards polyanionic cathode frameworks, which exhibit better structural stability with Na-exchange and high voltages. Some of the most explored polyanionic frameworks, such as the sodium superionic conductors (NaSICONs), alluaudites, olivines, and pyro/fluoro-phosphates display a wide variety of electrochemical performances, with low capacity being a common drawback. In this context, oxyfluorides are a largely unexplored class of polyanionic cathode materials, that can exhibit a high capacity and voltage, theoretically, with the voltage enhancement arising from the inductive effect of the fluoride ions. Here, I will present a systematic computational exploration of the transition-metal-based oxyfluoride chemical space, including finding the groundstate polymorph, evaluating the average Na-intercalation voltage, estimating the 0 K thermodynamic stability and quantifying the mobility of Na-ion mobility within the framework. Our work identifies a few promising compositions for subsequent experimental validation and paves the way for constructing NIBs with enhanced energy densities.
Moore-K1
Nanoionics and Iontronics are emerging disciplines dealing with the ionic transport properties at the nanoscale and the effect of a tuneable arrangement of ions on the electronic properties, respectively. These two disciplines try to understand and exploit the subtle interplay between electrons and ions and its application to innovative solid state-based devices to promote a revolution similar to the one driven by nanoelectronics few decades ago. In particular, since the main conversion and energy storage technologies are based on ionic, electronic or mixed-ionic electronic conductors (MIEC), these new disciplines are called to revolutionize the energy field by giving rise to entirely new and disruptive technologies [1,2,3].
In this talk, I will present our last advances in thin film oxides with ionic conduction for their application in solid oxide cells, lithium ion batteries and switchable devices for information technologies [4,5]. Moreover, I will pay special attention to the characterization of ionic transport phenomena in the nanoscale and for materials in operando conditions [6,7,8].
Moore-O1
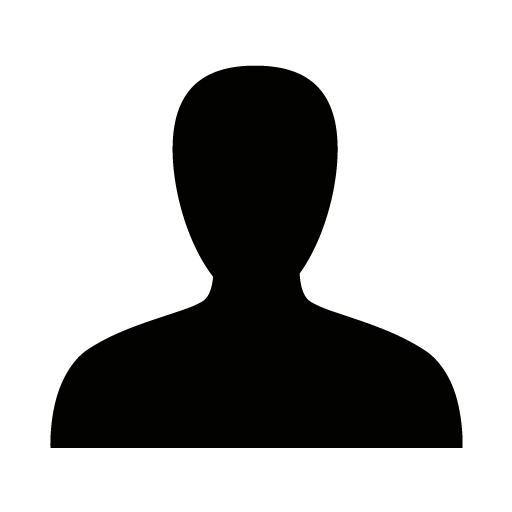
To develop efficient all-solid-state batteries, solid-state electrolyte separator need to meet several criteria, such as high electronic resistance and low ionic one, (electro)chemical, mechanical and thermal stability, and scalable processing.[1] As a thin-film, only the compounds from the oxide family meet these requirements. The most popular one, used in solid-state μ-batteries, is the lithium phosphate oxynitride (LiPON), thanks to its stability against lithium metal and its low electronic conductivity.[2, 3] However, its low ionic conductivity in the range of 10-6 S/cm, and its low deposition rate during reactive RF magnetron sputtering restrict its scale-up for industrial applications.
In this work, we use a carbon-doped Li3PO4 sputtering target to enable faster deposition rates. The high electronic conductivity of the target allows us to use a power density up to ~15 W/cm2, thus increasing the deposition rate threefold in RF mode and tenfold in DC mode, as compared to the standard RF mode of LiPON, while maintaining comparable electrochemical properties. Secondly, we investigate a Si- variant of LiPON, known as LiSiPON[5], by co-sputtering Li3PO4 and Li4SiO4 in reactive nitrogen gas to further improve the ionic conductivity. Finally, hybrid cells are fabricated to demonstrate the potential application of these separators in bulk solid-state batteries, consisting of composite cathodes, sputtered thin-film separators, and evaporated lithium metal anode.
Moore-O2
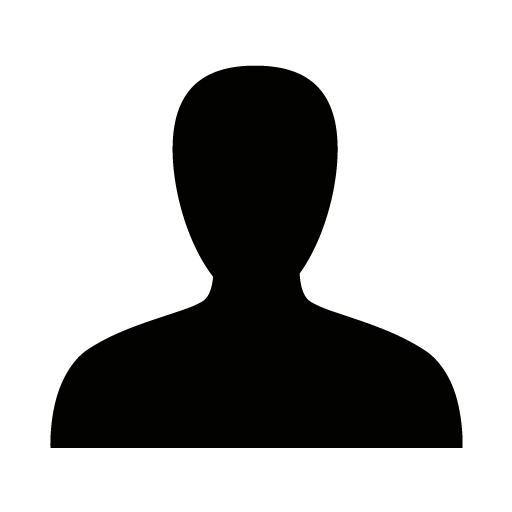
Thin film solid state Li-ion batteries have shown great promise for next generation energy storage devices. Their reduced dimensions, larger gravimetric specific capacity, rapid charge/discharge rates and long cycle life make them an attractive alternative to conventional battery technologies. Moreover, they possess additional benefits in the form of improved operational safety and compatibility with microfabrication technologies [1].
The current commercially available thin film solid state Li-ion batteries possess metallic Li as an anode, which limits their safety and environmental stability, thereby requiring it to be sealed from the environment.
Moreover, current technology allows only the use of amorphous LiPON as the thin film electrolyte because other materials show insufficient conductivity when processed as thin films. This not only limits the possible choices of electrode-electrolyte couplings but also preclude high temperature fabrication of the SSB heterostructure which is required for the growth of the electrodes but would lead to a crystallization of the LiPON layer.
Herein, we evaluate the suitability of an all-oxide Li-ion battery fabricated by pulsed laser deposition. For this purpose, we have employed Li4Ti5O12 (LTO) as the anode, LiMn2O4 (LMO) as the cathode and Li4-xGe1-xPxO4 (LGPO) as the electrolyte. LTO exhibits a promising anodic electrochemical performance, with a stability window between 0 V and 5 V , as well as a low volumetric change during Li (de)intercalation [2, 3]. LMO is known for its non-toxicity, high redox potential and specific capacity (4.2 V and 145 mA.h/g) which makes an attractive cathodic layer candidate [4]. As a solid-state oxide electrolyte alternative to LiPON, LGPO exhibits a high ionic conductivity (up to 10-6 S/cm) at room and high temperature (500 oC) regardless of its crystalline properties [5].
We investigate the structural, morphological and electrochemical properties of LTO and LMO, grown on Nb-doped SrTiO3 substrates buffered with La-doped BaSnO3 as a current collector in order to provide an improved matching of the lattice parameters and thereby reduce interfacial strain. Both layers are shown to grow epitaxially and can be cycled in liquid cells at high C rates (12C for cathode and 100C for anode). Moreover, LGPO thin films are confirmed to possess Li ion conductivities in the previously reported range.
Subsequently, we demonstrate the potential for the growth of a three-layer structure consisting of LTO, LGPO and LMO to form a complete thin film solid state Li-ion battery and demonstrate the capability for growth on Si substrates to make the fabrication of the complete heterostructure compatible with the conventional microfabrication methods.
Abbey-K1
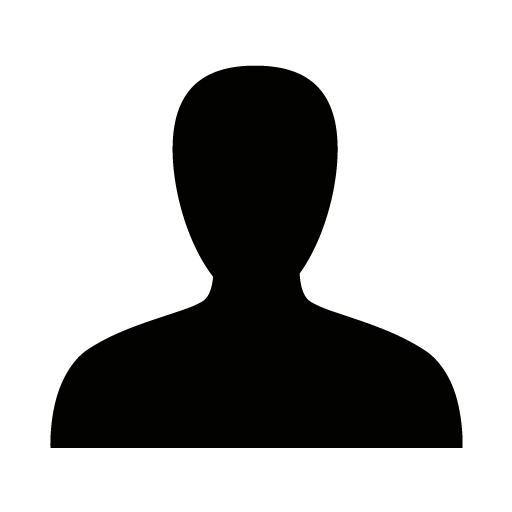
Lithium ion and oxygen ion based electrochemical cells are of outstanding importance in solid state ionics, and particularly lithium ion batteries (LiBs) but also solid oxide cells (SOCc) have gained tremendous relevance for a more sustainable energy economy. Interestingly, the concepts to investigate and interpret materials properties in such Li+ or O2- based devices are often different, despite all the similarities of the fundamental atomistic processes. For example, defect chemical considerations are very common for SOC materials, but rarely used for LiB materials. Chemical storage of a formally neutral species, on the other hand, is key in LiBs but much less applied in SOCs; only very recently an oxygen ion battery (OiB) has been introduced, based on voltage driven oxygen insertion into oxides [1].
In this contribution, the so-called chemical capacitance [2] is shown to be a measurable quantity which is highly useful for interpreting and understanding materials properties in both “worlds” of solid state ionics, in the Li world as well as the oxygen world. First, the meaning of the chemical capacitance in mixed conducting materials is recapitulated, which often gives a direct measure of minority charge carrier concentrations. In the next step, it is demonstrated how the chemical capacitance can be determined by means of impedance measurements and data analysis with transition line models. Then, the dependence of the chemical capacitance on the exact electrode composition (charging state) and its defect chemical interpretation are used to understand measured electrode potentials and thus charge-discharge curves of battery materials in both “worlds”. In the case of LiBs, it is shown that, for example, already the consideration of site restriction in defect chemical models allows us to understand peaks of measured chemical capacitances and thus shapes of charging curves of spinel-type cathodes (e.g. LiMn2O4) [3]. Also the relation between the chemical capacitance and the Li chemical diffusion coefficient is discussed. For OiBs, on the other hand, the chemical capacitances are related to Brouwer-diagrams of electrode materials (e.g. (La,Sr)FeO3, (La,Sr)(Cr,Mn)O3, SrTiO3) and subsequently connected to the electrode potentials of these materials, when being used as electrodes in OiBs. Thus, chemical capacitance measurements can help identifying promising novel electrode materials. Finally, it is shown that also oxygen in the gas phase can act as a chemical capacitor which exhibits a peak when increasing the chemical potential due to activity reasons [4].
Abbey-O1
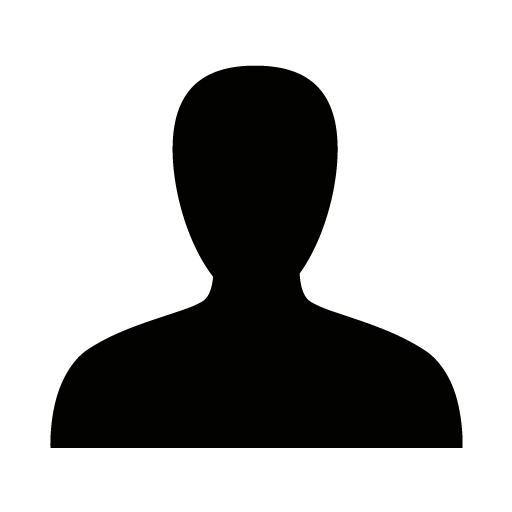
Mixed ionic electronic conducting (MIEC) oxides are frequently studied as electrode materials for solid oxide fuel or electrolysis cells. However, their ability to change stoichiometry depending on the oxygen chemical potential also enables charge storage typical for battery electrodes: By applying a current, formally neutral oxygen in the form of oxide ions and electron holes can be incorporated or released, thereby annihilating oxygen vacancies and creating electron holes or vice versa. This reversible electrochemical oxidation or reduction of the MIEC oxide can be exploited for energy storage, similar to lithium intercalation in lithium ion batteries.
In this contribution, such oxygen ion batteries are experimentally realized and extensively tested with different electrode materials. Thin film electrodes were grown by pulsed laser deposition on yttria stabilized zirconia single crystal electrolytes and sealed to inhibit oxygen exchange with the atmosphere. The charge–voltage characteristics of difference MIEC oxide electrodes — La0.6Sr0.4FeO3-δ (LSF), La0.6Sr0.4CoO3-δ (LSC), La0.9Sr0.1CrO3-δ (LSCr) and La0.5Sr0.5Cr0.2Mn0.8O3-δ (LSCrMn)— were studied by galvanostatic DC measurements at 350 to 500 °C, revealing specific electrode capacities up to 350 mAh/cm³ at half-cell potentials between +0.1 and -0.85 V vs. 1 bar O2 and excellent coulomb efficiency and cycle stability. LSCrMn electrodes even allow reversible charging down to voltages of ca. -2 V with capacities > 1000 mAh/cm3 Operando electrochemical impedance spectroscopy and in-situ synchrotron X-ray absorption spectroscopy were employed to investigate the defect chemical processes during charging and discharging of the electrodes, revealing the close relationship between the MIEC oxide defect chemistry (Brouwer diagram) and the charge–voltage characteristics of the corresponding electrode: Specifically, the MIEC oxide’s reducibility determines the electrode half-cell potential and its dopant concentration governs the electrode capacity. Electrodes with different reducibilities of the transition metal cation thus allow operation of oxide ion batteries. Full cell oxygen ion batteries with LSF cathodes and LSCrMn anodes were fabricated and operated at 350 to 400 °C with electrode related energy densities up to 250 J/cm³ (70 mWh/cm³), coulomb efficiencies >99 % and less than 1 % capacity loss after 100 charge cycles.
Abbey-O2
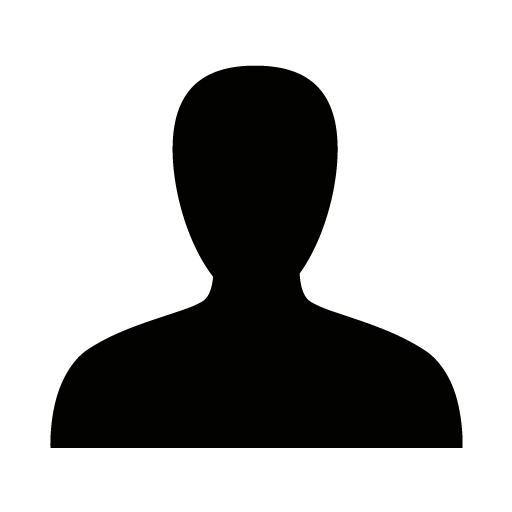
Amorphous SiO2 is promising anode material for next generation lithium-ion batteries (LIBs) that could potentially provide both high specific capacities, a theoretical capacity of 1965 mAh·g-1, and excellent cycling stability [1]. Diatom frustules have been shown to be a sustainable source of naturally nanostructured SiO2 which can be used in anode fabrication, displaying capacities of 800 mAh·g-1 after 100 cycles [2], [3]. However, SiO2 undergoes a complicated array of reactions at multiple potentials during lithiation resulting in the formation of electrochemically active silicon dispersed in a complex matrix of electrochemically inert lithium silicates and lithium oxides [4]. The formation of these irreversible phases is often linked to the low initial columbic efficiencies of SiO2 anode materials, as large reservoirs of lithium are trapped irreversibly. Electrochemical activation of SiO2 material is also crucial for electronic and ionic transport properties which improves after initial lithiation [5], [6]. However, driving forces and reaction pathways for SiO2 lithiation are not well understood due to the amorphous nature of lithiation reaction products, and it is imperative to fully grasp the mechanisms behind these reactions to utilize SiO2 anodes to their full potential. Improved knowledge on the evolution of the conversion reactions would for example aid the selection of the most appropriate SiO2 nanostructure as well as the appropriate cycling parameters.
To study the reaction mechanisms behind SiO2 lithiation, electrodes consisting of 75 wt% active SiO2 diatom frustule, 15 wt% carbon black conductive additive and 10 wt% of sodium alginate binder were assembled into 2032 half-cells with lithium metal as the counter electrode. The electrodes were cycled to different potentials in the first two lithiation/delithiation cycles and were subsequently held at that potential for 48 hours. This potentiostatic step was introduced to ensure the establishment of an electrochemical equilibrium within the electrode material. Chemical state analysis of both the electrode surface and buried interphases at different states of charge and cycle number were obtained by X-ray photoelectron spectroscopy (XPS) and Hard X-ray Photoelectron Spectroscopy (HAXPES, BM25 ESRF) respectively. High-resolution Transmission Electron Microscopy (TEM) and Selected Area Electron Diffraction (SAED) characterizations were also conducted on these samples for more accurate identification of the lithium silicate phases as well as to provide insight on the spatial distribution of the lithiation products.
Using a specially designed in-operando cell set-up, X-ray total scattering data in conjunction with computed tomography data were collected during initial SiO2 lithiation using hard X-rays (DESY). This allowed for real time tracking of SiO2 lithiation reaction and transition to amorphous phases, complementing therefore results from XPS, HAXPESH and TEM.
Preliminary result analysis clearly indicates the formation of lithium silicates in conjunction with pure silicon species after SiO2 lithiation. An increase proportion of SiO2 lithiation products can be found closer to the particle centers, indicating a reaction mechanism with lithiation beginning from the center of the SiO2 particles which propagates outwards towards the surface of the particles. This can help explain differences in SiO2 nanostructures with respect to their efficiency toward silicon formation, and also provide guidelines for identification of the most suitable diatom frustules.
Gielgud-K1
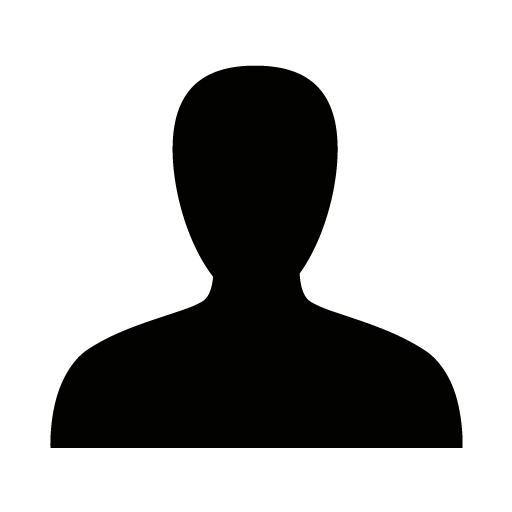
Protonic ceramic electrochemical cells (PCECs) are solid-state electrochemical devices using the proton-conducting oxide as the electrolyte. Besides power generation as fuel cells, PCECs is an emerging technology that could be employed for flexible chemical manufacturing by using a range of feedstocks. Economically competitive PCEC systems have distinct advantages over their counterparts at lower or higher temperatures, but further technology development and widespread market acceptance will require continuous innovation of materials and structures in order to improve cell performance, enhance system lifetime and reduce cost. Herein, we report the advancement of PCEC with new electrode component, catalyst integration, unique electrode structures as well as deep investigation on the state-of-the-art electrolyte materials to enable high-performance and robust operation for chemical and fuel production when sustainable feedstocks (e.g. steam, carbon dioxide and nitrogen) are used. Special emphasis is placed on how the operating temperature is pushed down to a lower region (e.g. 350-500oC), which shows huge potentials of PCEC as process intensified and decarbonization technologies compared with those industrial mature processes. At the end, we will also highlight INL’s effort on advanced manufacturing and scaleup of PCEC.
Gielgud-O1
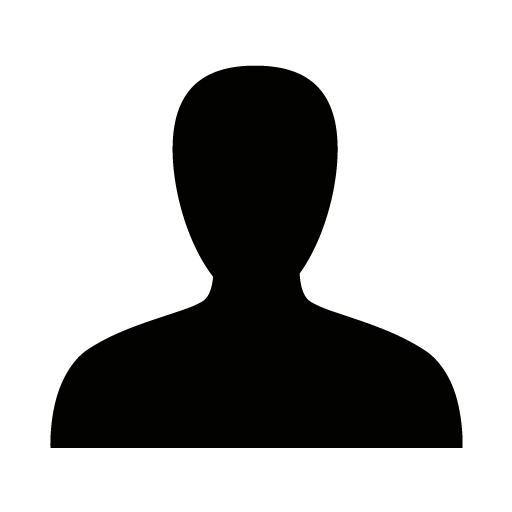
Catalytic membrane reactors (CMR) based on H2-separation membranes can be used to shift the equilibrium of thermodynamically-limited reactions, improving their performance. Industrial processes such as high-pressure steam methane reforming, ammonia cracking, non-oxidative aromatics production, and water gas shift reaction (WGS) could be benefited by these membranes.[1] In these industrial processes, the membrane surfaces are typically exposed to steam, CO2, CO, H2S, and hydrocarbons in combination with high temperature, requiring for the membrane materials to be long-term thermo-chemically stable in the mentioned conditions. Stability and operability in H2S presence is of outstanding importance since many of the membrane ceramic materials are surface poisoned and decomposed even in the presence of several ppm of H2S. Here, we have assessed and characterize the influence of H2S on the crystalline structure, lattice composition, surface chemistry and hydrogen transport properties of La5.4WO11.1−δ (LWO), one of the reference protonic membrane materials.
The LWO material was submitted to different gas cycles and treatments composed of i) sulfur-free humidified 10% H2 in N2 (2.5% H2O) and ii) humified 10% H2 with several concentrations of H2S (⁓2000 and 4000 ppm) in N2. Measurements were performed from 400 to 700 °C at atmospheric pressure. XRD, XPS, FESEM, WDS, EDS, and FIB-SIMS analyses demonstrated the incorporation of sulfide ions in the crystal lattice, as well as allowed to quantitatively determine how they are distributed among the bulk and the surface. The formation of La2O2S was also highlighted after the treatment at 700 °C. The substitution of oxygen by sulfur in the crystal lattice promoted changes in the transport properties. UV-vis spectroscopy and EIS measurements illustrated the enhancement of the electronic conductivity mediated by the concurrent partial reduction of tungsten cations (W6+), confirmed by XPS. The rise in electronic conductivity led to an increase of the H2 permeation, which has reached a flux of 0.042 mL cm−2 min−1 at 700 °C for a ∼770 mm-thick membrane, in contrast with negligible H2 permeation in H2S-free conditions.[2]
These findings show the sufficient stability of LWO membranes under H2S atmospheres, which could stand peaks of H2S concentration at intermediate temperatures without losing their integrity, circumventing the need of feed stream purification. They also introduce the possibility of tuning the ambipolar conductivity by incorporating sulfide anions in the LWO oxygen sublattice.
Fleming-K1
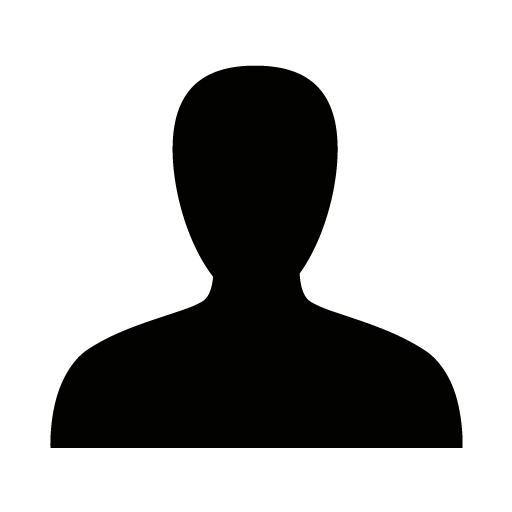
Solar thermochemical hydrogen (STCH) production uses concentrated sunlight to produce hydrogen using reduction/oxidation of metal oxides. Typically, the metal oxide is heated to high temperature (>1400 oC) causing it to release oxygen, then it is cooled to a lower temperature (~<1000 oC) in steam whereby it re-oxidizes, stripping oxygen from water molecules and producing hydrogen. Due to improved stability, non-stoichiometric oxides that do not change phase during the STCH process are typically used, even though the reversible oxygen content is less than phase changing materials. HydroGEN (h2awsm.org) is an Energy Materials Network supported by the U.S. Department of Energy’s Hydrogen and Fuel Cell Technologies Office, and is focused on the development of new water splitting materials for STCH by fostering collaboration between U.S. national laboratories and external entities such as academia and industry. In this presentation, recent progress in developing new water splitting materials will be presented. STCH performance of select materials predicted from a defect Graph Neural Network trained on crystal structures derived from density functional theory, subsequently synthesized and characterized using thermogravimetric analysis and flow reactor measurements, will be presented. Additionally, there will be discussion of benchmarking metrics and characterization of exemplar STCH materials. Lastly, trends in water splitting materials and durability considerations and challenges for commercialization will be discussed.
SNL is managed and operated by NTESS under DOE NNSA contract DE-NA0003525
Fleming-O1
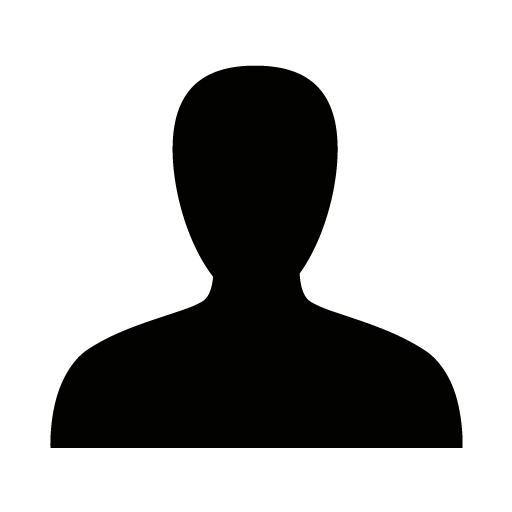
The industrial production of ethylene (C2H4) is central to modern societal applications such as plastics, epoxides and synthetic rubber. However, the commercial process involving the steam cracking of ethane/naphtha is the second-biggest CO2-generating process in industry.1 Thus, novel synthesis routes are necessary to decarbonize industrial C2H4 production. In 1982, Keller and Bhasin first reported on an alternative C2H4 production method, the oxidative coupling of methane (OCM),2 which can directly convert CH4 into C2H4. Unfortunately, this OCM approach is limited by significant “deep oxidation” where CH4 is instead combusted to CO/CO2 and H2O. To improve the C2 (C2H4 and C2H6) selectivity and yield of OCM, recent works have integrated OCM activity into solid oxide electrolyzers. In this electrochemical OCM (EOCM) approach, O2− ions from the cathode are transported to the anode where they oxidise CH4 to yield the desired C2 products. While the initial results are promising,3 little is known about the fundamental surface chemistry which contributes to the selective CH4 to C2H4 conversion in these systems.
In order to study the mechanistic details of selective CH4 activation, herein we use La0.3Sr0.7TiO3 as a mixed ionic-electronic conducting anode for EOCM. Combined electrochemical and gas chromatographic analyses demonstrate that this anode can successfully yield the desired C2 products and can respond to changes in the O2− flux. In particular, we illustrate that the C2 selectivity can be tuned using the applied potential: we show an unprecedented tuneability in the C2 selectivity with enhancements of >3x at higher current densities relative to values obtained at lower current densities. These increases in the C2 selectivity are then correlated with the onset of oxygen evolution at the anode surface. Thus, we hypothesize that by coupling CH4 activation to the oxygen evolution reaction, it is possible to selectively produce the active oxygen species which are necessary for selective CH4 activation towards the C2 products. These results furnish an understanding of how to increase both the CH4 conversion and C2 selectivity and have finally broken the inverse relationship between the CH4 conversion and the C2 selectivity that has traditionally limited thermochemical OCM approaches.
Fleming-O2
Jeong Woo Han is currently an associate professor in the Department of Materials Science and Engineering at Seoul National University (SNU). He also holds an Editor position of Molecular Catalysis published by Elsevier and an Associate Editor position of Korean Journal of Chemical Engineering publish by Springer. Before joining SNU in 2023, he was a full professor in the Department of Chemical Engineering at Pohang University of Science and Technology and an assistant/associate professor in the Department of Chemical Engineering at the University of Seoul. He obtained his Ph.D. at the Georgia Institute of Technology in 2010 and was also a postdoctoral associate at MIT until 2012. His current research area is computational modeling of catalysis and energy materials such as automotive catalysis, fuel cell electrodes, liquid organic hydrogen carriers, single atom catalysis, and so on.
Ammonia, a compound composed of nitrogen and hydrogen, is rapidly emerging as a novel hydrogen carrier. Its potential in fuel cell technology is increasingly recognized due to its high hydrogen density, ease of liquefaction, and established transportation infrastructure. Such advantages of ammonia have naturally led to the development of Direct Ammonia Solid Oxide Fuel Cells (DA-SOFCs). Despite these prospects, current research on DA-SOFCs faces critical limitations, particularly in catalyst selection. Ru, widely known for its high catalytic activity in ammonia decomposition, is a precious metal, thus raising economic concerns due to its high cost. This presents a significant challenge in the practical application and widespread employment of DA-SOFCs. Therefore, exploring alternative catalysts that can offer comparable activity to Ru at a lower cost is urgently needed.
Another significant challenge lies in the choice of promoters. For ammonia decomposition catalysts, their activity is widely known to be strongly enhanced by promoters. However, in the high-temperature and humid conditions of fuel cells, commonly used alkali and alkaline earth metal promoters are unstable and thus cannot effectively working as promoters. Moreover, while oxides or the oxygen vacancies within these oxides, are known to potentially act as promoters, their effectiveness in enhancing catalytic performance is limited. Therefore, there is also urgent needs to develop stable and effective promoters for DA-SOFCs.
Herein, we systematically designed a low-precious-metal, high-performance DA-SOFC system through DFT calculations. Our approach divided the DA-SOFC system into two components: the metal catalyst and the promoter. We analyzed the requisite properties for each component to achieve high activity and designed appropriate catalysts and promoters. For the metal catalyst, nitrogen adsorption energy was selected as a descriptor to design an alloy catalyst with superior performance to Ru. NiFe alloy was found to have a nitrogen adsorption energy very close to Ru at a certain composition (Fe = 50~75%). Furthermore, NiFe exhibited a lower activation energy than Ru in the N2 associative desorption reaction, confirming its potential as an outstanding catalyst to replace Ru. For the promoter, the work function was selected as a descriptor to analyze electron donation capability. To ensure both superior electron donation capability and stability, we developed a strategy that involves intrinsically doping the oxide support with a donor element. This approach effectively integrates the support itself as a promoter, addressing the stability issues posed by conventional promoters in fuel cells. Nb-doped STO (NSTO) was chosen as a donor-doped oxide and found to have a work function below 1.5 eV, which is much lower than that of conventional promoters and any oxide with oxygen vacancies, demonstrating that doping oxides with donors not only produces stable promoters but also highly effective ones.
To evaluate the performance of the NiFe-NSTO system designed through DFT calculations, NiFe-STO and NiFe-NSTO cells were fabricated, and ammonia conversion test was conducted. Both NiFe-STO and NiFe-NSTO showed a high conversion rate confirming the superior performance of the NiFe catalyst. Additionally, NiFe-NSTO outperformed NiFe-STO across all temperature ranges from 500 to 700℃, validating that the doped donor acts effectively as a promoter in harsh fuel cell environments. Our research may guide the further development of highly stable and active fuel electrodes for high-performance SOFCs and suggest that donor doping in oxides is an effective strategy for producing and maintaining promoter effects in fuel cells.
Fleming-O3
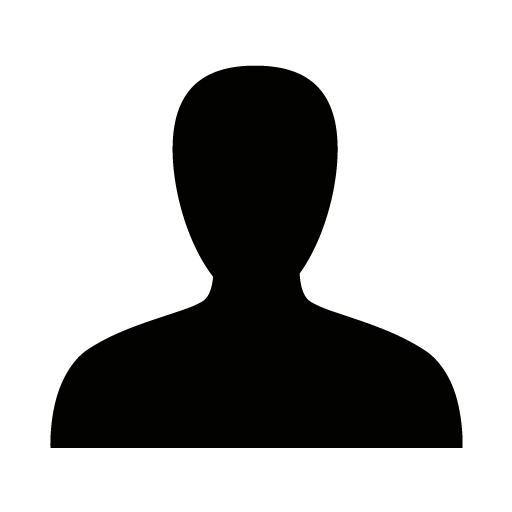
SrTi0.5Mn0.5O3 (STM55) perovskite has been proven experimentally to be a promising material for hydrogen production using thermochemical water splitting . However, optimizing the performance of STM55 in this application requires synergistic study of its thermochemical properties using both experiments and computations. In particular, there are several questions with regard to its crystal structure, magnetic ordering, electronic structure, and more importantly its off-stoichiometry variation as a function of temperature and oxygen chemical potential. Herein, we tackle these questions employing density functional theory calculations equipped with onsite Hubbard (U) terms applied to Mn and Ti 3d states. Since prior studies did not convincingly determine best values for UTi and UMn, we decided to systematically vary these Hubbard terms between 0 and 5 eV in steps of 1 eV to understand their effect on the properties of STM55. Our analysis revealed that UMn has a more dominant impact on the properties of STM55 in comparison to UTi. Higher UMn decreases the oxygen vacancy formation energy and hence the oxide becomes more reducible. More importantly, we found out that UMn = 1 eV is the best in computing oxygen off-stoichiometry of this oxide in comparison to prior experiments. However, the temperature dependence of the off-stoichiometry is not well-reproduced by our DFT calculations. Further analysis showed that mere reliance on temperature-independent formation energies of oxygen vacancies is not sufficient to correctly reproduce the temperature dependence of the off-stoichiometry. This hints to the importance of including thermal effects in the DFT study of this compound. In addition to this, our study showed that STM55 behaves as a metal (regardless of the ingredients of the DFT calculations), and is best modelled as a ferromagnet in the pm3m crystal structure. We also predicted the enthalpy and entropy of reduction of this oxide based on an Arrhenius-type analysis. While our model is capable of predicting the temperature dependence of these quantities, the UMn that leads to the best agreement with experiments is different than the one that best describes the off-stoichiometry. This led to the conclusion that there is no unique set of Hubbard parameters that can completely describe the behavior of STM55. Our work furnishes the grounds for further studies on this oxide, especially kinetic studies. Additionally, our results have general implications for the class of metallic oxides.
Westminster-K1
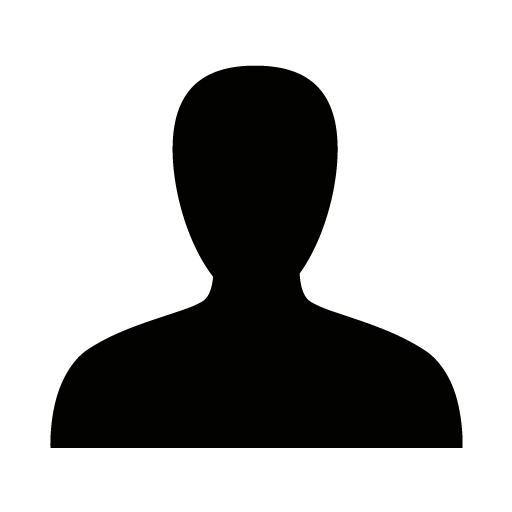
Screening with molecular simulations, and more recently with machine learning, is establishing an amazingly effective computational toolkit for materials discovery. In this talk I describe some of our recent work demonstrating that molecular simulation-based screening can discover new materials with outstanding oxygen kinetics mediated through both vacancy (e.g., BaFe0.125Co0.125Zr0.75O3-d)[1] and interstitial (e.g., La4Mn5Si4O22+d)[2] mechanisms. We have also shown that the ability to form the relatively less common interstitial oxygen diffusers depends on simple criteria of electron availability and structural flexibility. We believe that these criteria can form the foundation for new approaches to discovery of novel interstitial oxygen diffusers.
I then share some of our recent work on machine learning methods for predicting oxygen kinetics, with a focus on Area Specific Resistance (ASR) in solid oxide fuel cell electrodes. We demonstrate that,[3] for properly cleaned ASR data, machine learning predictions based on simple elemental properties are quite good (MAE ≈ 0.2 log units). Using a temporal cross-validation scheme we show that these machine learning models can be used for effective materials discovery. We also demonstrate that our ASR models are as good or better than correlations with traditional ab initio descriptors like oxygen p-bands, while being orders of magnitude faster to apply. Finally, we demonstrate that emerging data extraction methods based on large language models[4] may greatly reduce the time needed to develop databases for constructing these types of machine learning materials property models.
Westminster-O1
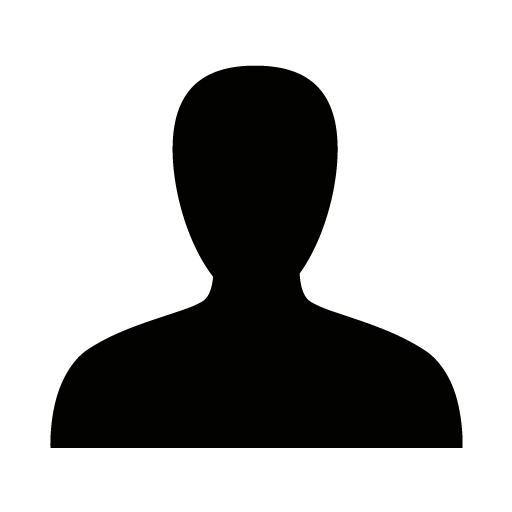
Hydrogen is an environmentally friendly energy carrier. The production of hydrogen, as well as hydrogen usage. should be accomplished using environmentally sustainable methods to achieve its ultimate objective. Water electrolysis, which produces hydrogen by decomposing water with the use of power generated from renewable energy sources, has gained recognition for its efficacy and has been continuously developed. It exhibits an inherent issue that the anode reaction, oxygen evolution reaction (OER), has sluggish reaction kinetics and necessitates a large voltage. Consequently, there is a requirement for efficient catalysts that can facilitate OER. Meanwhile, replacing OER with a biomass upgrading reaction that requires less operating voltage and generates high-value products is receiving great attention. An example is ethanol oxidation reaction (EOR) which produces acetate by oxidizing ethanol. However, unlike OER, there is little research translating the catalytic performance to the electronic structure.
Perovskite oxide is a promising, non-platinum-group metal catalyst due to its structural stability, excellent catalytic activity, and compositional and structural flexibility. Specifically, substituting an aliovalent element in the A-site can improve its performance by indirectly changing the states of B-site transition metals and oxygen ions, which actually affect the electrochemical activities. In OER field, several studies have been reported on substituting Sr2+ for La3+ in LaCoO3, but there is a lack of research on substituting Ca2+. Specifically, there is no research probing fundamental material properties to unravel the exact effect of Ca substitution to catalytic performance. Furthermore, it has solely been applied to conventional water electrolysis reactions.
Here, we highlight the effect of Ca substitution in the A-site of LaCoO3 perovskite for OER and EOR in alkaline electrolytes. The perovskite series of La1-xCaxCoO3-δ (x = 0, 0.1, 0.2, 0.3, 0.4, 0.5, and 1) possessing both highly crystallinity and similar morphologies is successfully prepared, which enables an accurate study comparing the intrinsic substitution effect. Both OER and EOR activity gradually increases with Ca substitution due to a facilitated charge transfer. With an increase in Ca concentration, the crystal structure symmetry is enhanced, and holes are created on the oxygen ligand and Co 3d t2g orbitals. These modifications increase the overlap of Co 3d and O 2p orbitals and create hole states, respectively. The DFT calculation result verifies that the changes ultimately lead to an electronic structure that is active in electrocatalysis. Beyond phenomena previously reported, further DFT calculation unravels that partial Co atoms in the perovskite oxide undergo a transition in their spin state; from an intermediate spin state to a high spin state with a down-spin orientation. We suggest, for the first time, that Ca substitution in LaCoO3 triggers the activation of the spin state of local Co atoms, thereby enhancing the electrocatalytic activities. This study is believed to offer a framework for translating electrochemical catalysts based on their electronic structure and spin state. Furthermore, it has the potential to provide new insights into the design of EOR catalysts.
Westminster-O2
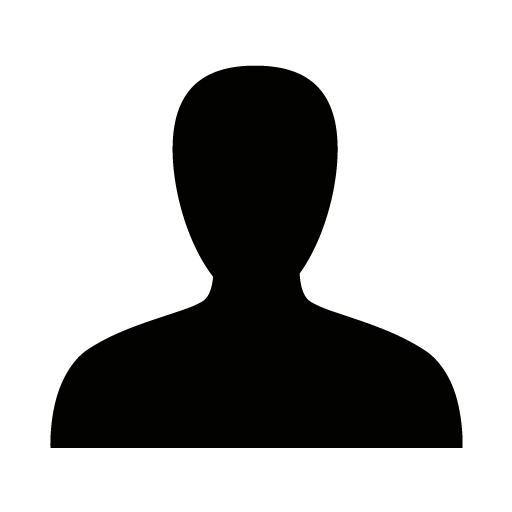
There is an urge to correctly understand the motion of ions under large electric fields due to implications in several technologies such as resistive switching memories, capacitors, and flash sintering. In spite, of over more than 70 years of analysis to this problem, a fundamental understanding is still missing. Herein, we conducted molecular dynamics simulations on two model oxide systems; a simple dielectric (CeO2) and a ferroelectric phase (f-HfO2) under a range of temperatures and electric fields. From these simulations, we extracted activation barriers for the “effective” diffusivities of the oxide ion and found out that in both oxides, the barriers can be correctly predicted using recent analytical models. These models are the ones that take into account local atomic polarization effects. Beyond this analysis, we tried to answer the question of the possibility of separating the drift from the diffusion contributions to the field-enhanced ionic motion. This was conducted by analyzing the time and space density correlation function of the oxide ion. We found out that complete decoupling of diffusion from drift is not possible and eventually one has to live with a “drift-mixed diffusion” or a “diffusion-mixed drift”. As an alternative approach to describe the motion of ions under electric fields, we analyzed the cumulants of motion up to the fourth cumulant. Our analysis revealed that these cumulants do not vanish indicated a highly non-Gaussian behavior for the motion of ions under electric fields. Even more problematic, higher cumulants (third and fourth) do not lend themselves to simple Arrhenius behavior. Our work does not completely address the needed understanding of field-enhanced ionic transport. But it furnishes the ground for future studies that can build on our analyses.
Westminster-O3
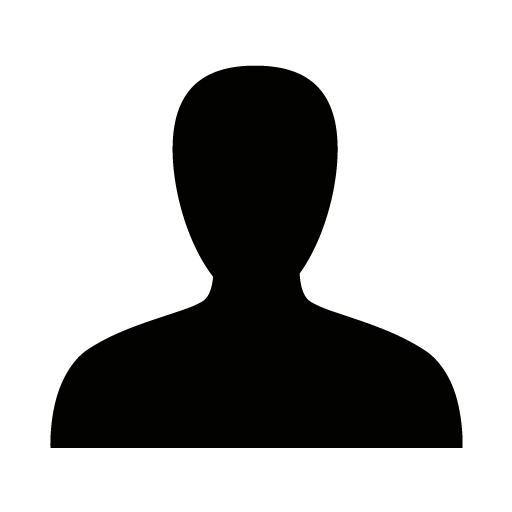
Biomass, a form of renewable energy, can be converted into methane through anaerobic fermentation[1][2]. Dry Reforming of Methane (DRM) reaction can be induced by Ni nanoparticles on CeO2 catalyst. In this study, we focused on the methane dissociation reaction (CH4→CH3-+H+), which is considered to have the high activation energy in the DRM reaction process[3].
To understand the reaction mechanism within the methane dissociation reaction, we found that behavior of hydrogen ion of methane plays important role to reduce the activation energy by density functional theory. Simultaneously, the DRM reaction was experimentally performed using Ni supported CeO2 flowerlike-catalyst and the activation energy of methane dissociation was calculated from the methane consumption rate.
The resulting experimental value for the methane dissociation reaction was 0.69 eV (15.91 kcal/mol) and the DFT value was 0.80 eV (18.45 kcal/mol). Both values were very close, confirming the validity of our calculations. This result indicates the validity of this study for future catalyst design with dopants in CeO2. These results provide a deeper understanding of the reaction mechanism and factors governing the activation energy of methane dissociation reactions and provide clues for effective catalyst design for the DRM reaction.
St.James-K1
Professor Emma Kendrick, CChem FIMMM FRSC FIMMM - Chair of Energy Materials, School of Metallurgy and Materials, University of Birmingham.
Prof Kendrick’s career to date has included industrial and academic roles leading to her current role as Chair of Energy Materials, where in addition to group lead of the energy materials group (EMG), she is co-director of the Centre for Energy Storage (BCES) and part of Birmingham Energy institute (BEI) and Birmingham Centre for Strategic Elements and Critical Materials (BCSECM). The EMG investigates sustainability in novel battery technologies from materials, manufacturing, performance and parameterisation, and recycling. Her recent work has led to a 2021 joint UoB - Imperial College London (ICL) spin out company, based around the methods of experimental parameterisation of applied multi-physics cell models, called About:Energy, for which she is founder and director.
Prior to UoB, she spent two years as Reader in WMG, University of Warwick. Before academia, she led innovations in the battery industry, latterly as Chief Technologist in Energy Storage at SHARP Laboratories of Europe Ltd (SLE) and prior to that for two lithium-ion battery SMEs, Fife Batteries Ltd and Surion Energy Ltd.
She is fellow of the Royal Society of chemistry (RSC) and Institute of Metals, Mining and Materials (IoM3). Recently, she has been recognised through several awards; 2021 Faraday Institution (FI) Researcher Development Champion, RSC 2021 Environment, Sustainability and Energy Division Mid-Career Award, and the 2019 Hothersall Memorial Award for outstanding services to Metal Finishing.
Prof Kendrick holds a PhD from Keele University, obtained as part of a postgraduate transfer partnership (PTP) scheme with CERAM Research, a MSc in new materials from the University of Aberdeen and a BSc in chemistry from the University of Manchester.
Critical materials are those which have a supply chain risk and are of high economic importance, this means that criticality is unique to each area of the globe, due to the sourcing of these different materials. In Europe, the critical materials list published in 2023 shows lithium, graphite, phosphate and phosphorous, silicon, cobalt and manganese, all classified as critical.1,2 Nickel is although, a strategic material, and with carcinogenic implications is not currently classified as critical. This assessment is dynamic due to changes in the sourcing, supply and also recycling levels of these important elements and materials. With the new battery passport and EU battery directive regulations, 50% of lithium recovery by 2027 and 80% by 2031 from spent lithium-ion batteries. SLI and EV batteries will require a recycled content of 16% for Co, 6% Li and 6% Ni, and recycling efficiency is 50% by 2025. Environmental and social impact needs to be understood throughout the life cycle of the battery, with supply chain due diligence. By 1st of Feb 2028, a full impact assessment of battery life cycle, or LCA must be supplied, including the recycled content sources.3,4
In this work the consideration of this impact is discussed, the assessment of criticality and the LCA for new battery technologies, and the development on new materials for lithium-ion and sodium-ion batteries.5,6 The circular economic approach to battery development is described, with advances in cathode developments and stability for improved manufacturability and recyclability.7–9 When developing new battery technologies, there's a chance to design in recycling from the start, rather than retrospectively, as is currently the case for lithium-ion batteries. Additionally, this presentation will explore design principles for creating a more sustainable battery technology, such as optimizing materials processing, electrode design, and properties that promote long-lasting performance and increased recovery efficiencies.
St.James-O1
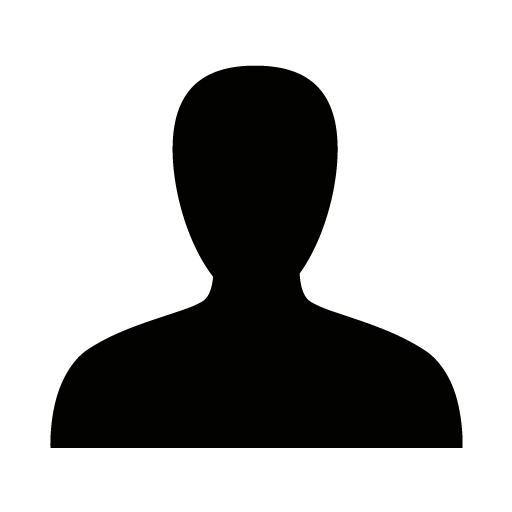
Integration of intermittent renewable energy sources demands the development of sustainable electrical energy storage systems. The abundance and low cost of sodium (Na) make Na-ion batteries promising for smart grids and grid-scale applications. In search of electrodes for Na-ion batteries, layered Na-based oxides with the general composition of NaxTMO2 (TM: transition metal) have attracted significant attention for their high compositional diversity that provides tunable electrochemical performance for electrodes in Na-ion batteries.
Compared to lithium (Li)-based layered oxides of the well-known LiCoO2 and Ni-rich LiNiyCozMn(Al)1-y-zO2, a striking difference is that for Na-ion oxides in addition to O-type, also the P-type stacking can occur, where P-type refers to prismatic Na-ion coordination. These stackings show distinctly different electrode performance, where the most studied layered stacking configurations are P2 and O3 types, referring to the ABBA and ABCABC oxygen stacking, respectively. P2-type oxides usually provide higher Na-ion conductivity and better structural integrity against the O3 analogues, which is responsible for the high-power density and good cycling stability. In search for electrodes with good chemical/dynamic stability and high Na storage performance, various layered oxides have been synthesized and investigated. However, effective guidelines towards the design and preparation of optimal electrode materials are lacking.
Here, we introduce the “cationic potential” that captures the key interactions of layered materials, and makes it possible to predict the stacking structure. Aiming at a simple descriptor for layered oxides, we express the extent of the cation electron density and its polarizability, normalized to the ionic potential anion(O), by defining the following equation:
Фcation=ФTMФNa/ФO
The distinct P2 and O3-type regions indicate that the cationic potential is an accurate descriptor of the inter-slab interaction, and thereby the structural competition between P2- and O3-type structures. A larger cationic potential, implies stronger TM electron cloud extend and interlayer electrostatic repulsion resulting in the P2-type structure, with more covalent TM-O bonds and an increased NaO2 slabs. Opposing this, a larger average Na ionic potential, achieved by increasing Na content, increases the shielding of the electrostatic repulsion between the TMO2 slabs, favouring the O3-type structure. This is demonstrated through the rational design and preparation of layered electrode materials with improved performance. As the stacking structure determines the functional properties, this methodology offers a solution towards the design of alkali metal layered materials.
St.James-O2
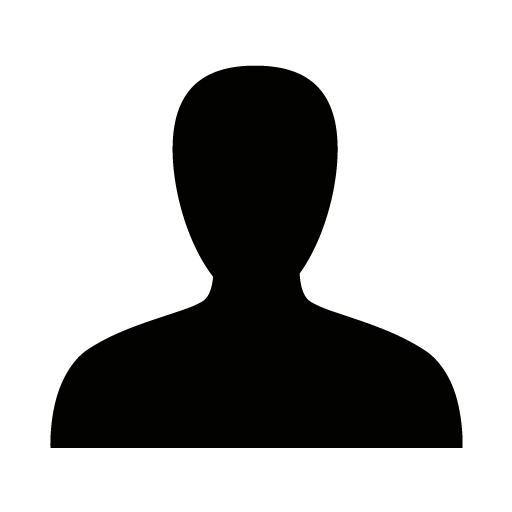
The rich redox chemistry of vanadium oxides has meant that they have been and continue to be the subject of exhaustive study in many areas and particularly in energy materials. High energy densities reported for vanadium oxides are related to the electrochemical activity associated with multiple V(V) to V(III) redox state changes. The large variety of stable and metastable structures are a challenge to synthesize vanadium oxides with high purity and controlled stoichiometry.
V4O9 is an intermediate crystal phase along the line from V2O5 to VO2. It is halfway in composition, although not structurally since it is not directly related to them; it is not a “shear structure” but a 3D tunnelled structure instead. Defined as a metastable phase, it has been little investigated. The use of reducing agents either in solvothermal conditions or in solid-solid or solid-gas reactions requires an accurate control of reaction conditions and usually leads to mixed phases.
In this sense and taking advantage of the exceptional catalytic properties of vanadium oxides, we have successfully prepared flake-shaped nanoparticles of V4O9 from nanoparticulate V2O5 and acetonitrile as solvent/reducer by a direct novel solvothermal method, which has proven to be very efficient and versatile. The as-prepared sample consisted of 5–20 nm average size V4O9 nanoparticles exhibiting an average oxidation state of 4.65 vanadium ions as deduced from XPS. The enhanced electronic conductivity of V4O9 is reflected in its electrochemical performances. Against Li anode upon discharge to 1.5 V, the V4O9 could intercalate ~6 Li+ corresponding to 464.8 mA h g-1 at 10 mA g-1 and exhibited reversible Coulombic efficiency of 75.2% upon charging to 4.0 V [1]. The V4O9 displayed superior rate capability at various current densities for 110 cycles and excellent long cycle stability delivering a reversible capacity of 160.8 mA h g-1 at 100 mA g-1 even after 2000 cycles. Preliminary electrochemical results against Na anode showed that V4O9 could intercalate 4 Na+ with highest first discharge capacity of 318.9 mA h g−1 and delivered a reversible capacity of 55.9 mA h g-1 at 250 mA g–1 even after 10000 cycles, being the first to report for sodium cells. The investigation of charge-storage mechanisms [1] showed that the electrochemical performance of V4O9 is surface controlled at very high rate but can still be considered an intercalation material when used at lower or medium rate providing a total high capacity. Of special interest for V4O9 application is that it stands very long cycling making it a challenging/ competitive cathode with high capacity and cyclability to replace expensive electrode compounds
St.James-O3
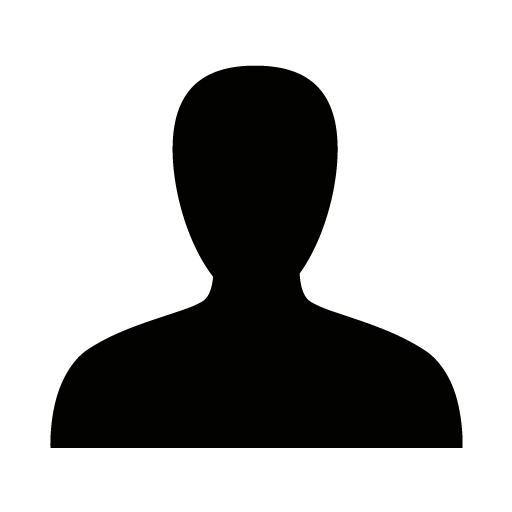
A subset of polyanionic salts of Li+ and Na+ exhibit crystalline phases with distinct rotational disorder of the polyanion that is typically commensurate with increased ionic conductivity of the cation. These mesophases are sometimes referred to as “plastic crystals” or “rotor phases” and combine properties of classical solids and classical liquids. Macroscopically they retain their shape and exhibit sharp Bragg diffraction ("solid-like") while locally they are dynamically disordered and exhibit high atomic mobility ("liquid-like").
The behavior of three such systems will be presented and contrasted, namely the “classical” Li2SO4 and Na3PO4, along with the recently discovered Na3PS4. [1,2] Classical crystallographic approaches (i.e. Rietveld refinement to diffraction data) either break down or provide limited information on the average structure without capturing the dynamics of such structures. Thus, attempts to complement the study of said systems via advanced crystallography (e.g. total scattering), spectroscopy (e.g. Raman, NMR, INS) and computations (e.g. molecular dynamics) will be presented.
The current understanding of how the polyanionic rotational diffusion might be correlated with the cationic translational diffusion (“paddle-wheel” effect etc.) will be discussed.
Moore-K1
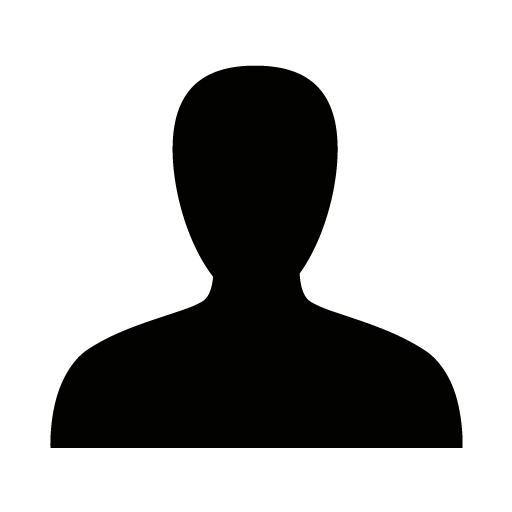
The brownmillerite phase of strontium cobaltite (SrCoO2.5) is an insulating antiferromagnet while the perovskite phase (SrCoO3) is a ferromagnetic metal. Relatively small changes to the oxygen concentration can drive a reversible, topotactic phase transition, making SrCoO3-δ a material of interest for ionotronic applications. While the electronic and magnetic properties of such oxides have been subjects of considerable interest, much concerning the kinetics and dynamics of oxygen vacancies remain unknown.
With the coherent X-rays available at the Advanced Photon Source, we can monitor in-situ the behavior of oxygen vacancies in epitaxial SrCoO3-δ thin films and bilayers grown on both SrTiO3 (001) and (LaAlO3)0.3(Sr2TaAlO6)0.7 (001). This allowed studies of oxygen migration in and out of the cobaltite under different conditions, providing insight into the kinetics and dynamics of oxygen-induced phase evolution in complex oxide heterostructures. From X-ray photon correlation spectroscopy performed at the brownmillerite superlattice reflection, we find that the oxygen vacancy dynamics is sensitive to epitaxial strain and electric field strength. We will discuss the kinetics and dynamics of the oxygen vacancy ordering transition and the methods used to distinguish the different mechanisms taking place.
Moore-O1
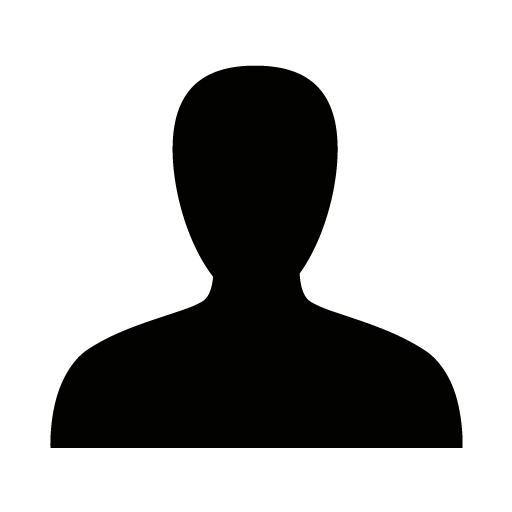
All-solid-state lithium batteries (ASSLBs) are considered as one of the most promising technologies for future energy storage, owing to their safety and high power density. NASICON-structured lithium aluminum titanium phosphate (Li1.3Al0.3Ti1.7(PO4)3, LATP) can be an enabling electrolyte for these devices owing to its high ionic conductivity and superior stability at high voltages (e.g. enabling LiCoO2 cathodes). Although it has not received much attention from a manufacturing standpoint due to insufficient electrochemical stability at low voltages (e.g. vs. Li anodes), it is promising for bilayer or multilayer electrolyte architectures. Our study focuses on the manufacturing of high-performance multilayer electrolytes, implying that our proposed micro-battery system includes an anode, anode-side electrolyte, possible interlayer, cathode-side electrolyte, and cathode.
We manufacture LATP thin films via a custom glovebox-attached pulsed laser deposition (PLD) system and study the fabrication parameter – stoichiometry – structure – conductivity relationships. To control the composition and address the tendency for loss of multiple light and/or volatile species, we use a multilayer growth approach from two targets, LATP and Li3PO4. By varying the LATP-Li3PO4 deposition ratio, the PLD deposition pressures, and heat treatment during or post-deposition, we study how those parameters affect 1) Li and PO4 concentrations by nuclear reaction analysis (referenced to heavier ions’ concentrations by Rutherford backscattering spectrometry), 2) crystallinity, and 3) structural (crystallographic phase) composition of the films, by grazing-incidence X-ray diffraction. The differences in the chemical and structural parameters are then correlated with ionic conductivity measured via in-plane electrochemical impedance spectroscopy (EIS) as a function of temperature in an inert environment. Our study identifies challenges and solutions for the manufacture of thin-film electrolytes containing multiple volatile species and light elements via PLD and provides understanding of how material phases and stoichiometry influence ionic conductivity.
Moore-O2
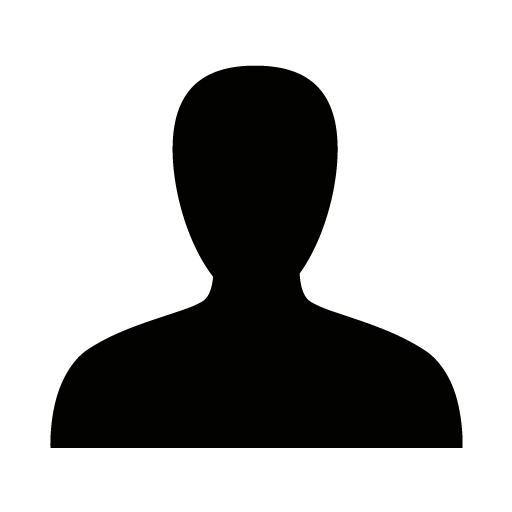
Since the early 1980s, thin film solid ionic conductors have been identified as a key component for miniaturised all-solid-state sensors and batteries [1]. Among them, amorphous Li-based materials prepared by vacuum deposition techniques are particularly attractive as electrolytes for making lithium microbatteries, and integrating the latter into smart autonomous microsystems. In that respect, lithium phosphorus oxynitride, prepared by reactive sputtering of a Li3PO4 target under a pure nitrogen atmosphere, exhibits a combination of interesting properties such as an ionic conductivity of ~2.10-6 S.cm-1 at room temperature [2], a very low electronic conductivity < 10-14 S.cm-1, and passivating behaviour on lithium metal, which led to the market launch of first microbatteries. But since then, energy density specifications for IoT and medical applications have led to changes in conventional cell design with more stringent requirements for ionic conductivity.
Amorphisation and then partial nitridation of lithium orthophosphate, leading to the formation of a disordered material containing condensed units with bridging nitrogen [3], was found to be an effective means of boosting ionic conductivity. The introduction of a second glass former, such as in Li-Si-P-O-N, can also provide an additional tenfold increase through a mixed former effect [4]. This evolution illustrates the need for research into materials that are more and more complex in their composition, and not confined to ‘simple’ systems, to identify the best ionic conductors. In this respect, high-throughput experimentation is an attractive approach for screening multi-element compositions [5]. It usually involves a combinatorial synthesis method capable of rapidly producing a large number of samples with different compositions, i.e. material libraries, combined with automated characterisation methods that allow various specific properties to be rapidly measured on all the samples produced.
The development of a specific experimental methodology for the high-throughput screening of thin film solid electrolytes based on the synthesis of material libraries by magnetron co-sputtering on 4’’ wafers and the rapid characterisation of their thickness (stylus profilometry), composition (LIBS, ICP-OES), structure (Raman spectroscopy) and conduction properties (Impedance spectroscopy) will be presented. Mapping the composition of these thin films containing low-Z elements, particularly Li, on large substrates is undoubtedly the most difficult stage in this process. Laser-induced breakdown spectroscopy (LIBS) appears to be the most appropriate technique for achieving this objective, as it meets the key requirements of speed, spatial resolution and sensitivity. However, additional developments are needed to achieve elemental quantification. To this end, combined ICP-OES, LIBS and ion beam (RBS, NRA) analyses were carried out.
Abbey-K1
Yet-Ming Chiang is Kyocera Professor in the Department of Materials Science and Engineering at MIT, where his research focuses on clean energy technologies including non-aqueous and aqueous batteries for transportation and grid-scale storage, and electrochemical production of industrial materials. He has published over 330 scientific articles and holds over 100 issued U.S. patents, of which more than 70 have been licensed to or are held by practicing companies. Chiang is a member of the U.S. National Academy of Engineering and Fellow of the Electrochemical Society, Materials Research Society, American Ceramic Society, and the National Academy of Inventors. His work in energy has been recognized by the TIME 100 Climate award (2023), the World Economic Forum’s Technology Pioneer Award (2016), the Economist’s Innovation Award (Energy and Environment Category, 2012), The Electrochemical Society Battery Division’s Battery Technology Award (2012), and an R&D 100 Editor’s Choice Award (2006). Chiang has brought several laboratory discoveries to commercialization, including high-power lithium iron phosphate batteries, the semi-solid electrode approach to low-cost lithium-ion battery manufacturing, and batteries for long-duration grid storage. He has co-founded several companies based on his research including American Superconductor Corporation (1987), A123 Systems (2001), 24M Technologies (2010), Desktop Metal (2015), Form Energy (2017), Sublime Systems (2020), and Propel Aero (2023). He co-directed the MIT Future of Energy Storage study (2022) and is co-director of the Center for Electrification and Decarbonization of Industry at MIT.
The stability of the metal – solid electrolyte interface during metal stripping and plating under wide-ranging, dynamic conditions is central to the performance of all-solid-state and hybrid rechargeable batteries utilizing a metal anode. Successful design of this anode-electrolyte subassembly would enable adoption of a wide variety of cathodes and cell chemistries. Previous work [1,2] in our group showed that dendrite propagation through a solid electrolyte couples electrochemistry to fracture, and is therefore responsive to imposed stress. Indeed, the growth of a metal dendrite can be “steered” by applied stress, including being deflected to avert shorting or, rather counterintuitively, being driven towards the opposing electrode if compressive stack pressure is applied, promoting short-circuit failure [2]. In this talk, results will be presented that shed light on the mechanism by which metal dendrites grow under electrochemical bias, obtained using a newly developed birefringence microscopy technique [3] to quantitatively map the stress field associated with propagating dendrites. These operando experiments combined with cryogenic scanning transmission electron microscopy (STEM) characterization of the dendrite tip allow us to distinguish the separate roles of electrochemistry and mechanics associated with dendrite growth in solid electrolytes.
Previous work [4,5] has also shown that greater stability, as measured by the critical current density (CCD) that can be sustained without closed-circuit failure, and the capacity (thickness) of metal that can be electrodeposited without open circuit failure, are both correlated with mechanically softer metal electrodes (e.g., Li vs Na vs K, or use of solid-liquid two-phase alloys). Pursuing this strategy, we show that deliberate incorporation of an immiscible soft phase into Li metal composite electrodes with suitable microstructures can both increase CCD and narrows its cell-to-cell variation, increasing reliability. Prospects for extending this approach to the cathode-solid electrolyte subassembly will also be discussed.
Abbey-O1
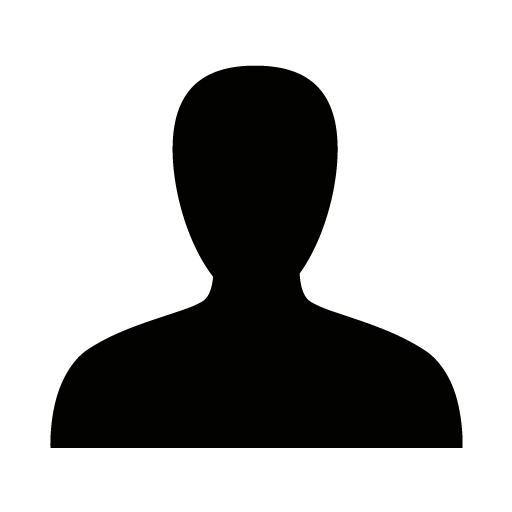
Solid-state electrolytes, encompassing inorganic solid electrolytes (ISEs), solid polymer electrolytes (SPEs), and gel polymer electrolytes (GPEs), are promising alternatives to liquid electrolytes with enhanced safety, stability, and the potential for compatibility with higher energy density devices.1, 2 Among these, GPEs offer distinct advantages, namely ionic conductivity similar to that observed in liquid electrolyte systems with the mechanical stability of polymer electrolytes. 3-5This study introduces a novel approach for the production of a GPE electrolyte from an initial SPE. The SPE, a blend of PEC (Poly (ethylene carbonate), HNBR (Hydrogenated butadiene rubber) and Li salt is produced without solvents via melt extrusion. Melt extrusion is a green manufacturing technique due to the absence of hazardous solvents and energy savings that result from avoiding lengthy drying steps.
The PEC phase of the resultant SPE is then decomposed in-situ via thermal treatment to yield EC, which is kept by the HNBR phase, to produce a GPE. The interaction between the polymer and solvent in the GPE significantly influences its electrochemical properties, particularly ionic conductivity, by improving electrolyte absorption and increasing Li+ ion mobility relative to the SPE. These interactions influence the properties of the GPEs including the temperature and time needed for SPE to GPE conversion.
Abbey-O2
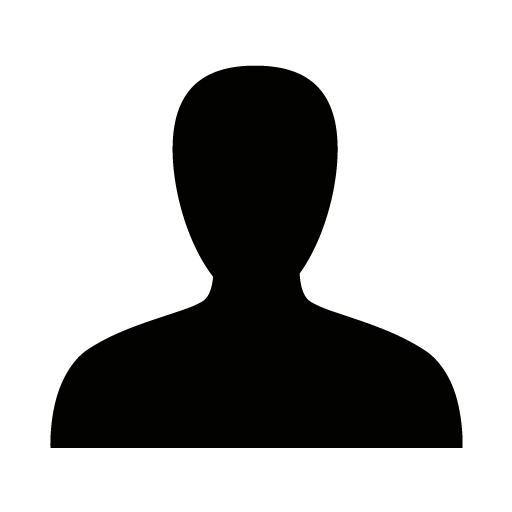
In the composite electrodes of the all-solid-state batteries (ASSBs), huge internal stress is induced by volume change of active materials during charge/discharge processes. Such mechanical stress triggers the capacity fading of the ASSBs and this phenomenon is severe for oxide-type solid electrolytes. In this study, we introduced stress buffers in the cathode composite layer of garnet-based ASSBs to reduce the stress. The cathode composite layer was prepared by quick liquid phase sintering[1] of LiCoO2 (LCO, Young’s modulus: 191 GPa) and Li6.5La3Zr1.5Ta0.5O12 (LLZT, Young’s modulus: 150 GPa) with a rather high loading of 7-8 mg-LCO/cm2. When no stress buffer was added, the charge/discharge capacity gradually decreased after dramatic initial drop. The addition of Li2.2C0.8B0.2O3 (LCBO, Young’s modulus: 50~80 GPa) or Li3BO3 (LBO, Young’s modulus: 100~110 GPa) as stress buffer successfully suppressed the polarization of the charge-discharge, and improved cycle-ability for the initial 10 cycles. However, the ASSBs with the stress buffers showed sudden capacity fading that is possibly caused by the lower fracture toughness of the stress buffers.
Abbey-O3
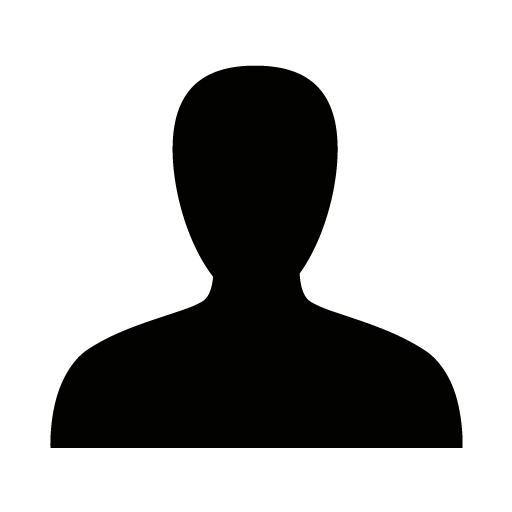
All-solid-state Li batteries exhibit significant potential for use as next-generation batteries. Sulfide solid electrolytes possess high Li-ion conductivity, making them suitable for all-solid-state Li batteries with a high-power density. For using the batteries in high-power applications, it is crucial to achieve a low interface resistance between sulfide solid electrolytes and positive electrodes. Thus, elucidating the origin of the interfacial resistance quantitatively to reduce the interface resistance is critical for the practical application of all-solid-state Li batteries.
There are two hypotheses regarding the origin of the interface resistance. First, an interphase layer is formed chemically or electrochemically. [1,2] Second, a space charge layer is formed at the interface. [3] The origin of the reduction in interfacial resistance remains debated despite intensive studies. It is well known that introducing a thin oxide layer, hereafter referred to as a “buffer layer,” reduces the interface resistance. Thus, studies on introducing a buffer layer, such as Li3PO4, LiNbO3, etc. are underway to reduce this interface resistance. However, the mechanism and the role of the buffer layers still remain unclear.
Accordingly, we utilized a thin-film model system with a clean interface, which can facilitate the measurement of the interface resistance and the quantitative analysis of the interface structure. [4,5] We previously developed a controlled interface using an amorphous Li3PO4 oxide solid electrolyte and LiCoO2 epitaxial thin-film positive electrode for quantitative analysis; the atomically well-ordered interface exhibits an extremely low resistance (~10 Ohm cm2). [6,7]
In this study, we quantitatively investigated the interface between an amorphous Li3PS4 sulfide solid electrolyte and a LiCoO2(001) epitaxial thin-film positive electrode and the effects of a Li3PO4 buffer layer on the interface resistance. When Li3PS4 contacts directly upon LiCoO2(001), the interface resistance exhibits a significantly high value (3.5 x 104 Ohm cm2), resulting in no battery operation. Scanning transmission electron microscopy (STEM) observation and X-ray energy dispersive spectroscopy (EDX) analysis revealed that a chemical reaction layer is formed as S diffuses from Li3PS4 to LiCoO2 before battery operation. In contrast, introducing a Li3PO4 buffer layer with 10 nm thickness reduces the interface resistance dramatically by ~1/2,800, leading to normal battery operation. STEM-EDX revealed that the Li3PO4 buffer layer suppresses the S diffusion from Li3PS4 into LiCoO2. Consequently, an abruptly sharp interface structure remains at Li3PO4 buffer layer-LiCoO2, exhibiting extremely low interface resistance. These results unveiled that the significantly high interface resistance between Li3PS4 and LiCoO2 originates from the formation of the chemical reaction layer, and the Li3PO4 buffer layer suppresses the S diffusion causing a chemical reaction. [8] This study paves the way for the strategically designed interfacial modification using the buffer layer between sulfide solid electrolytes and positive electrodes.
Gielgud-O1
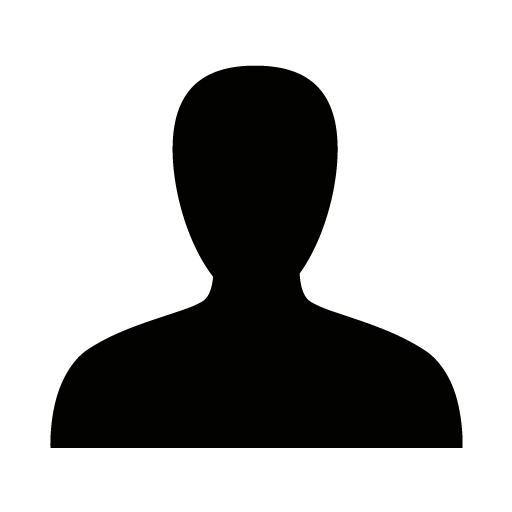
Fast charging and high power delivery in rechargeable battery electrodes requires rapid ionic and electronic transport. In addition, long-term cycling demands electrode stability and the reversibility of ion insertion and extraction. Two structural families that satisfy these criteria are the crystallographic shear structures and bronze-like phases that are found in many niobium-containing binary and ternary early transition metal oxides. Several members of these large families have demonstrated the ability to store large quantities of lithium at rates well above 1C up to and above 20C; these rates are accessible without nanostructuring.
The maximum power output and minimum charging time of a lithium-ion battery – key parameters for its use in, for example, transportation applications – depend on mixed ionic–electronic diffusion. While the discharge/charge rate and capacity can be tuned by varying the composite electrode structure, ionic transport within the active particles represents a fundamental limitation. Thus, to achieve high rates, particles are frequently reduced to nanosize dimensions despite this being disadvantageous in terms of volumetric packing density as well as cost, stability, and sustainability considerations. As an alternative to nanoscaling, we show that complex niobium oxides with topologically frustrated polyhedral arrangements and dense micron-scale particle morphologies can rapidly and reversibly intercalate large quantities of lithium. Multielectron redox, buffered volume expansion, and extremely fast lithium transport approaching that of a liquid lead to extremely high volumetric capacities and rate performance as reported in both crystallographic shear structure and bronze-like niobium-based oxides[1–6]. The active materials offer new strategies toward designing electrodes with advantages in energy density, scalability, electrode architecture/complexity and cost as alternatives to graphite and Li4Ti5O12.
Gielgud-O2
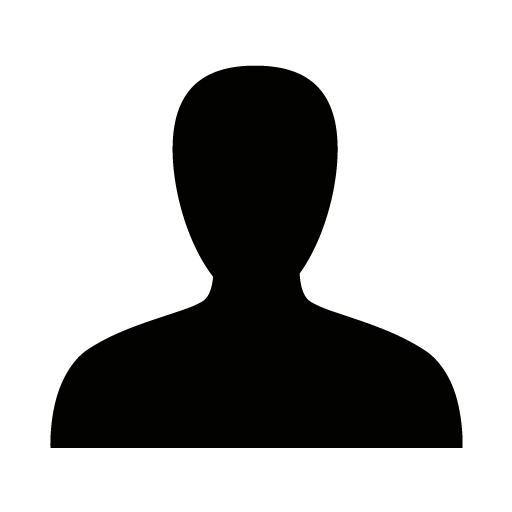
Composite electrodes are revolutionizing ceramic electrochemical cells, including both oxygen-ion and proton conductor types, by combining materials with complementary properties. This synergy improves the efficiency, durability, and overall performance of these electrochemical devices. A key innovation is the hybrid oxygen electrode made from a layered double perovskite and a single-layered perovskite. This combination enhances both the surface oxygen exchange and the bulk oxygen-ion diffusion, leading to better performance in both oxygen reduction and evolution reactions. Incorporating nano-micro composite structures further enhances these benefits. These structures combine nanoscale particles with microscale frameworks, improving ionic conductivity and mechanical stability. The synergy between nano and micro components facilitates efficient ion transport and robust structural integrity, even under high operational stress. The benefits of composite electrodes are clear. The composite electrode enhances both power generation and hydrogen production efficiencies while reducing the operating temperatures. The manufacturing process for these electrodes is straightforward and cost-effective, involving wet-chemistry synthesis and controlled calcination. Moreover, composite electrodes demonstrate exceptional stability under high current densities and extended use, proving their potential for long-term, efficient energy solutions. This advancement represents a major step forward in developing scalable, efficient, and sustainable energy technologies.
Gielgud-O3
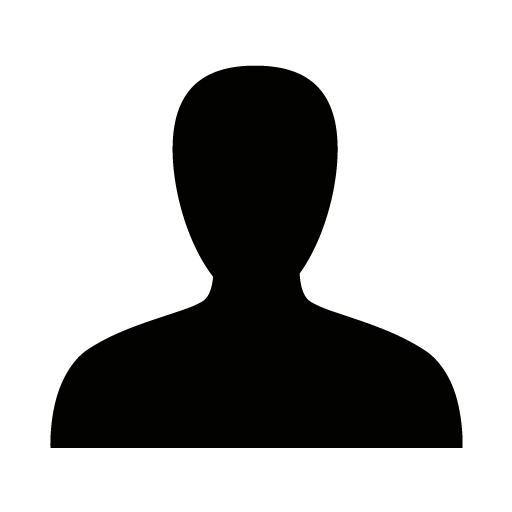
Predicting practical rates of transport in condensed phases enables the rational design of materials, devices and processes. This is especially critical to developing low-carbon energy technologies such as rechargeable batteries. For ionic conduction, the collective mechanisms, variation of conductivity with timescales and confinement, and ambiguity in the phononic origin of translation, call for a direct probe of the fundamental steps of ionic diffusion: ion hops. However, such hops are rare-event large-amplitude translations, and are challenging to excite and detect. Here we use single-cycle terahertz pumps to impulsively trigger ionic hopping in battery solid electrolytes. This is visualized by an induced transient birefringence, enabling direct probing of anisotropy in ionic hopping on the picosecond timescale. The relaxation of the transient signal measures the decay of orientational memory, and the production of entropy in diffusion [1]. We extend experimental results using in silico transient birefringence to identify vibrational attempt frequencies for ion hopping. Using nonlinear optical methods, we probe ion transport at its fastest limit, distinguish correlated conduction mechanisms from a true random walk at the atomic scale, and demonstrate the connection between activated transport and the thermodynamics of information.
Furthermore, we study the influence of this memory on the correspondence between descriptors extractable from atomistic simulation and macroscopically observable transport rates and activation energies. Using large-scale simulations, we reproduce the frequency dependence of AC ionic conductivity and of activation energies [2]. We show that due to the collective nature of ion transport single ion hops cannot be considered a step of macroscopic-derived random-walk models typically used to understand ion diffusion and conduction in condensed phases.
Gielgud-O4
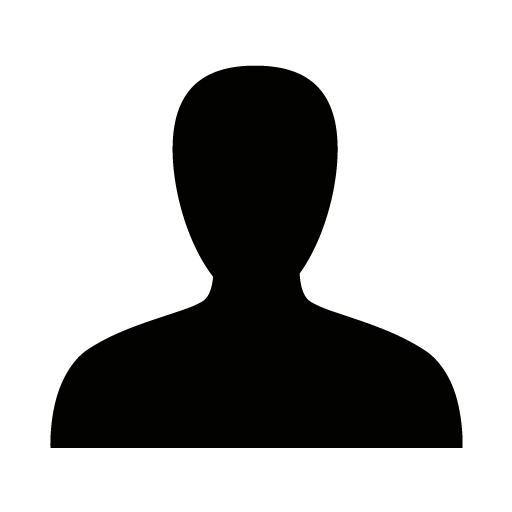
Ionic defects are essential building blocks for properties of complex oxides, including mixed ionic and electronic conductivity, surface kinetics and reactivity, lattice expansion and dynamics, which are important for applications ranging from energy (e.g., fuel cells and electrocatalysts) to information (e.g., memristors, neuromorphic computing devices). In this talk, I am going to cover two fronts of harnessing ionic defects for tailed oxide thin film properties. Firstly, to accurately predict electrochemical driving force needed for manipulating ionic defects for designed properties, constructing phase diagrams that correlates physical properties (conductivity, (chemical) diffusivity, lattice constant, etc.) and ionic defect concentration is essential. However, such tasks often require a large number of samples and can be susceptible to artefacts introduced by sample-to-sample variations. To tackle this challenge, we have developed a new electrochemical device that can introduce spatially-graded ionic defect concentrations in one single oxide thin film sample. Combined with materials characterization tools with high spatial resolution, we achieved high-throughput construction of phase diagrams controlled by ionic defect concentration [1,2]. Secondly, we established a comprehensive theoretical framework to understand the pivotal role of ionic defects (e.g., oxygen vacancies) in determining the activity of oxide electrocatalysts for both high-temperature [3] (>500°C, solid/gas interfaces) and low-temperature [4] (<100°C, solid/liquid interfaces) oxygen evolution reactions (OER). We found that, in both scenarios, alterations in ionic defect concentrations contribute significantly to what we refer to as “chemical overpotential”, which represents a substantial portion of the total overpotential. Overall, our work provides a holistic understanding of both “static” and “dynamic” effect of ionic defects in determining the properties and functionality of oxide thin films.
[1] H. Chen, Q. Lu* et al., Nano Letters, 22 (2022), 8983-8990
[2] Y. Lu, Q. Lu* et al., ACS Nano, 17 (2023), 14005−14013
[3] K. Yang, Q. Lu* et al., JACS 145 (2023), 25806–25814
[4] Y. Hu, Q. Lu* et al., in prep.
Gielgud-O5
Iwnetim (Tim) Abate is an Assistant Professor in the Department of Materials Science and Engineering at the Massachusetts Institute of Technology. His work combines electrochemistry, X-ray and electrochemical characterization with quantum mechanical simulations to design materials for decarbonization and sustainability. Prior to joining MIT DMSE, he was both a Miller and Presidential Postdoctoral Fellow at UC Berkeley working on layered materials (including 2D materials and heterostructures) for application in computing, catalysis, and sensing. He completed his Materials Science and Engineering Ph.D. at Stanford, with or under Prof. William Chueh and Prof. Thomas Devereaux. His PhD aimed at improving the energy capacity of Li- and Na-ion batteries to meet the ever-growing global demand for energy storage. Prior to joining Stanford, he did a research stint at IBM Alamden and Los Alamos National Laboratory working on metal-air batteries and hybrid perovskite solar cells, respectively. Iwnetim was born and raised in Ethiopia.
Outside the lab, Iwnetim is a co-founder of a non-profit organization (www.scifro.org) working on empowering the African youth to solve local problems through scientific research and innovation. The organization is generously supported by the Bill & Melinda Gates Foundation, National Science Foundation, American Physical Society and others.
Intercalation electrodes exhibit rich point defect chemistry with complex and dynamic local chemistry. Foundational to (electro)chemical and catalytic transformations in these electrodes are stable and reversible high-valent redox (HVR) couples. The "over" oxidation needed to create these cationic and anionic redox couples is strongly linked to structural disorder, resulting in vacancies, interstitials, Frenkel, and antisite defects. In this talk, using transition metal oxides (TM-O) as examples and combining computational and experimental methods, we report two major findings.
First, we developed a comprehensive mechanism and framework for understanding the source of the structure-redox coupling. [1,2] We discovered that during HVR, the highly oxidized species have a strong thermodynamic driving force to form short O-O covalent bonds to become stable. However, O-O dimerization induces significant and energetically unfavorable local bond strain, which is alleviated by point defect disorder, achieving overall energy savings. This disorder is mostly irreversible and leads to significant voltage hysteresis, preventing the harnessing of extra activity or capacity from HVR for (electro)chemical and catalytic applications in batteries, catalysis, and memory devices. Consequently, high-valent redox couples have been historically avoided.
Second, we demonstrate a mechanism by which structural disorder and voltage hysteresis can be completely avoided during HVR. [3,4] We discovered that the introduction of ordered TM point defects in intercalation materials during synthesis significantly changes the thermodynamic and kinetic energy landscape of defect formation during HVR. More specifically, the ordered cation vacancies provide an electrostatic templating platform that uniquely enhances the coulombic interaction between oxidized oxygen species and interlayer vacancies. This kinetically stabilizes localized O-hole polaron species and prevents O-O dimerization. In summary, our work provides an exhaustive understanding of the complex and dynamic defect chemistry in intercalation electrodes, underscoring the roles of covalent bonding, point defects, and electrostatics as critical governing factors and design principles.
St.James-K1
Philipp Adelhelm is a physical chemist and works at the interface between the research disciplines of materials science and electrochemistry. His current main interest is research on sustainable batteries.
After studying materials science at the University of Stuttgart, he moved to the Max Planck Institute of Colloids and Interfaces in Potsdam (Department of Prof. Antionetti / Smarsly, 2005-2007) for his doctoral project. This was followed by a 2-year postdoctoral stay at the University of Utrecht (Prof. de Jongh) and then a position as a junior research group leader at the Institute of Physical Chemistry of the Justus Liebig University in Giessen (Prof. Janek, 2009-2015). From 2015-2019 he was a professor at the Institute for Technical Chemistry and Environmental Chemistry at the Friedrich Schiller University Jena.
He has been a professor at the Institute for Chemistry at Humboldt-University since 2019 and heads a joint research group on operando battery analysis at the Helmholtz Zentrum Berlin (HZB).
Layered materials are the foundation of modern Li-ion battery technology and are also used in the Na-ion batteries currently entering the market.[1],[2] A very successful strategy for tuning the properties of layered oxides is to change their composition, e.g. moving from LiCoO2 to NMC or NCA chemistries. This approach, along with understanding the redox activity of oxygen, is also currently a hot topic for Na-ion batteries too. Understanding the effects of doping / chemical substitution of layered oxides is complicated and requires a variety of analytical tools including synchrotron-based methods as well as theory, for example[3],[4]. A second, much less explored strategy for tuning the properties of layered materials is the co-intercalation of solvent molecules. An excellent example is the intercalation of solvated Na-ions into graphite, leading to the family of so-called ternary (or quaternary) graphite intercalation compounds. The use of co-intercalation in electrode reactions opens up a very diverse field of research, but at the same time there are also challenges that can be studied using, for example, operando electrochemical microscopy and operando electrochemical dilatometry. The talk will also present a new model for the formation of these compounds, which is very different from previous assumptions.[5] Recently, the concept of solvent co-intercalation has also been demonstrated for a layered sulfide, suggesting that also cathode active materials may be tuned by co-intercalation.[6],7] Most of the results have been obtained through a Consolidator grant funded by the European Research Council (SEED). During his presentation, the speaker will also take a look back - and into the future - from the perspective of a mid-career professional.
[1] Sodium-ion batteries: Materials, Characterization and Technology ISBN: 978-3-527-34709-4, Wiley Dec 2022, Titirici/Adelhelm/Hu (Editors)
[2] P. Nayak et al. Angew. Chemie. Int. Ed., 2018, DOI: 10.1002/anie.201703772
[3] L. Yang et al. Adv. Functional Materials, 2021, DOI: 10.1002/adfm.202102939
[4] Y. Li et al., Adv. Materials 2024, DOI: 10.1002/adma.202309842
[5] G. Avall et al. Adv. Energy Materials, 2023, DOI: 10.1002/aenm.202301944
[6] G. Ferrero et al. Adv. Energy Materials, 2022, DOI: 10.1002/aenm.202202377
[7] Y. Sun et al, 2024, submitted
Fleming-K1
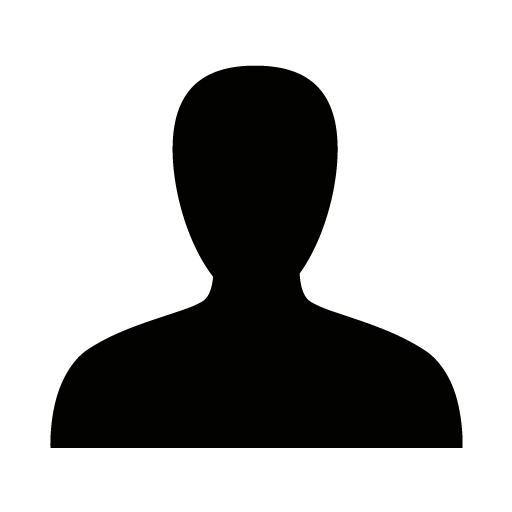
Materials with oxygen storage capacity can be used to make chemical processes more efficient and more environmentally benign. The original way of making hydrogen some 120 years ago was known as the steam-iron process. Iron oxide was reduced in a fuel gas to produce particulate iron. This iron was then oxidised in steam to produce hydrogen and magnetite. The cycle was then repeated. Such processes have the advantage of separating the products of the fuel oxidation from the hydrogen product leading to a greater thermodynamic efficiency and less need for further separation. The reactor functions in what is known as a 'chemical looping' mode whereby a bed of solid oxygen carrier is exposed to gaseous oxidising streams and reducing streams in a periodic fashion.
As a result of their inherent advantages, chemical looping processes are once again in the research spotlight. Of interest is oxygen carrier material design to fit the chemical process in question, oxygen carrier synthesis and oxygen carrier characterisation (particularly in-situ or preferably operando). Here we will discuss these aspects and refer to recent studies concerning the use of ‘exsolution’ to produce more active oxygen carrier materials [1] as well as the use of advanced characterisation techniques including in-situ TEM [2] and operando synchrotron-based XRD [3] and neutron scattering.
Fleming-I1
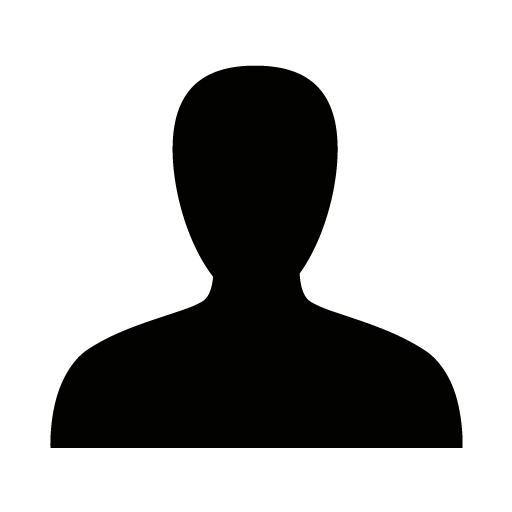
I will share two recent studies on ceria:(1) The band structure of ceria influences not only its conductivity but also its reactivity. Yet operando experimental studies investigating the correlation between the 4f band and surface reactivity are still rare. To bridge this gap, we developed synchrotron-based operando ambient pressure X-ray photoelectron spectroscopy relaxation. This enabled us to study the surface redox kinetics of atomically flat CeO2 ultra-thin films under different biaxial strains (-3.27% to 3.55%), by monitoring the f band while altering pO2 more than 10 orders of magnitude within a minute. We discovered that tensile strain significantly enhances surface redox kinetics. Ab initio calculations suggest that tensile strain reduces the energy barrier of the surface redox reaction and promotes oxygen vacancy formation. Moreover, tensile strain decreases the band gap of ceria by lowering the conducting band's energy level, thereby facilitating easier charge transfer. (2) Recently study found the acidity of surface-infiltrated binary oxides can act as a descriptor for oxygen exchange kinetics in mixed conducting oxides such as Pr-doped ceria. We performed operando quantitatively modulation of alkaline oxide on Pr-doped ceria surface, using an integrated system of pulsed laser deposition, RHEED, impedance, and a glovebox. We observed a 'volcano shape' in oxygen exchange kinetics as the amount of alkaline oxide increased, with peak performance being ten times better than in ex-situ experiments. We propose that the interface of alkaline oxide/ mixed conducting oxide significantly aids the formation of oxygen vacancies, thus enhancing oxygen exchange kinetics. We believe that these two studies will spur further development of in situ kinetic studies of mixed ion-electron conducting oxide materials.
Fleming-O1
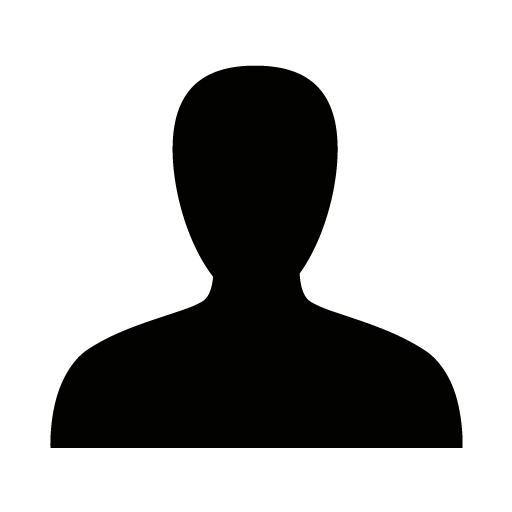
Miniaturized electrochemical devices are essential for supplying power to bio-electronic implants. Compared with traditional implantable batteries, glucose fuel cells (GFC) use glucose which is abundant in body fluid as the constant source of reactant, and offers a sustainable power solution without the need for replacement surgeries.1 Unlike glucose fuel cells with polymer electrolytes, inorganic solid-state materials and abiotic catalysts has the advantage of miniaturizability, improved long-term stability and power density.2 Our previous work based on ceria's high H+ conduction at ambient3,4 demonstrates the highest measured power densities to date and is fully compatible with standard semiconductor fabrication methods. This presents great potential for using it as the integrated and direct power source of bio-electronic devices and implants.
Expanding upon prior research, this study proposes the evolution of the existing proof of concept—a double chamber fuel cell with a ceria solid-state electrolyte and non-selective catalysts—into an implantable single-chamber fuel cell design. The novel design features side-by-side placement of electrodes in the same plane, situated on top of a ceria thin film functioning as a proton-conducting electrolyte. An insulating polymer membrane is incorporated to prevent short circuits in the wet environment of the human body. Throughout the investigation, our emphasis lies on fundamental research pertaining to proton conduction in ceria membranes and the identification of selective catalyst systems for the glucose fuel cell. Leveraging substantial concentration differences between coexisting glucose and oxygen in bodily fluids, we achieve predominantly kinetically controlled glucose oxidation and diffusion-controlled oxygen reduction, with high nanoscopic surface area electrodes showing heightened selectivity for glucose and lower surface area electrodes favoring oxygen. This work on proton conducting ceramic single chamber glucose fuel cells contributes to the exploration of energy-efficient and sustainable solutions for tracking and supporting diverse bodily functions within the realm of medicine.
Fleming-O2
Electrochemical CO2 reduction (eCO2R) is a very promising way to convert detrimental CO2 emissions into sustainable fuels and chemicals, and move us closer to a net zero or net negative carbon economy.1 To compete with traditional fuel/chemical production routes, electrocatalysts needs to be highly active, selective towards a desired product, and stable. Thanks to the use of gas diffusion electrodes (GDEs) that provide gaseous CO2 close to the electrocatalyst, high activities can be achieved.2,3 However, product selectivity as well as stability still need to be improved before applications. An emerging strategy to drive product selectivity and improve stability is to control the local environment surrounding an electrocatalyst, i.e., the concentration of the products and reagents therein.4
In this work, we achieve such control by tuning the mass transport of the eCO2R reactants, namely CO2 and H2O, to the reaction sites by varying pore size and hydrophobicity of polymeric GDE substrates coated with Ag or Cu electrocatalysts.5,6 We show that for GDEs with large pore size and weak hydrophobicity, a deeper penetration of water/ aqueous electrolyte takes place, yielding, in the Ag case, poor selectivity towards CO and a significant production of H2. In contrast, GDEs with small pore size prevent this local flooding of the electrocatalyst, thus yielding a greatly improved Faradaic efficiency towards CO (up to 95% at 100 mA/cm2 in neutral electrolyte), minimal H2 evolution, and remarkable long-term stability (97% of initial CO selectivity retained after > 40 h).5 In the Cu case, we observe a strong enhancement in C2H4 selectivity when employing GDE substrates with small pores and strong hydrophobicity (up to 50% Faradaic efficiency in neutral electrolyte at 200 mA/cm2 for > 3 h).6 Both CO2 and CO reduction experiments on Cu GDEs indicate the presence of mass transport limitations for the gaseous reactants in all GDEs, however this limitation is less severe for GDEs with small pore sizes. We rationalize these findings with the presence of an electrolyte layer covering the electrocatalyst, forcing the electrocatalytic reaction to take place at double phase boundaries. Under these conditions, CO2 mass transport limitations are governed by the extent of electrolyte penetration within the GDE and can thus be controlled by acting on the substrate.5,6 In addition to fundamental understanding, our results also provide a scalable strategy to improve product selectivity and stability of GDEs for eCO2R.
Westminster-K1
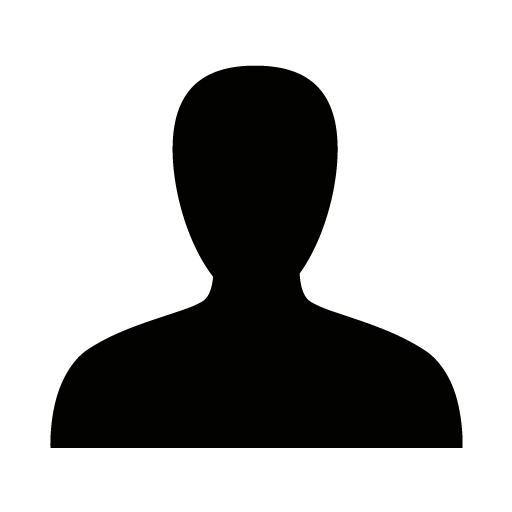
Mixed niobium based oxides are increasingly gaining research and commercial momentum as next generation battery materials. They generally present in the Wadsley-Roth (W-R) crystal structures consisting of a central block, with Nb in corner sharing sites and edge sites that adjoin neighbouring blocks. In particular, the transition of Nb (3+ --> 5+) oxidation states allow for high lithium intercalation. The niobium oxides can be partnered with other transition metals (TM) such as Vanadium (V), Phosphorous (P), Tungsten (W) and Titanium (Ti) among others, to avail a plethora of materials for exploration. Ranging in the size of block (3x3 --> 3x5 and mixed block systems) and varying oxidation states which result in increasing the ion storage capacity of these materials. Which makes them very attractive for battery applications.
In this keynote, several W-R materials will be discussed. Where materials modelling techniques have been used to better understand the influence of the transition metal reducibility on structural, electronic as well as transport properties. The talk will include a review of the literature as well as our most recent work, on several mixed niobium oxides.
Our recent work suggests the expansion and contraction of the blocks influences the volume expansion as Li intercalates. The electronic structure calculations give insights on the reducibility of the transition metal cations while the dynamical simulations suggest favourable pathways and the influence of the TM on the transport properties.
Westminster-I1
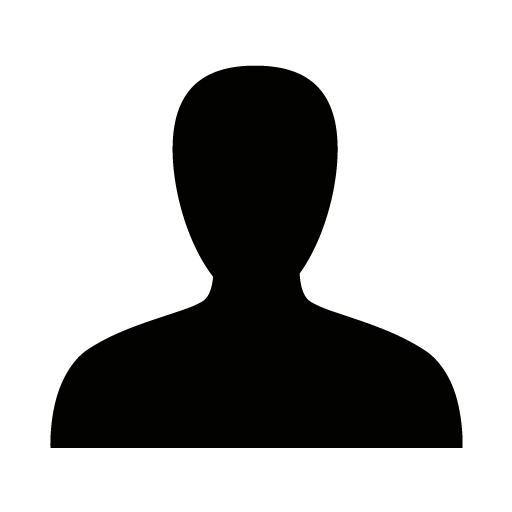
DFT is applied to study the mechanism of oxygen dissociation on the SrO-terminated surfaces of strontium titanate and iron-doped strontium titanate [1]. Our study reveals that while O2 dissociation is not favored on the SrO-terminated perovskite surface, oxygen vacancies can act as active sites and catalyze the O–O bond cleavage. Electron transfer from lattice oxygen atoms to the O2 molecule, mediated by the subsurface transition metal cations, plays an important role in the resulting formation of surface superoxo species. Our focus on the SrO-terminated surface, rather than the TiO2 layer, which is presumed to be more catalytically active, was driven by experimental observation using low energy ion scattering spectroscopy, which reveals that the surface of SrTiO3 after high temperature heat treatment is SrO-terminated. The interaction of SrO terminated SrTiO3 surface with molecular carbon dioxide and water has been investigated using first-principle theoretical methods and surface analysis techniques. We have studied the formation of a surface SrCO3 layer and various possible products of H2O interaction with the SrO surface, such as, surface chemisorbed water and the formation of a surface hydroxide layer [2]. The co-adsorption of CO2 and H2O was explained both theoretically and experimentally showing that its products follow a complex temperature dependence and as a result, the surface composition may vary between carbonate and surface chemisorbed water. Our theoretical simulations have shown that the presence of water molecules in the gas phase might assist the molecular oxygen/lattice oxygen exchange reaction by stabilization of the surface oxo species in the transition state with a hydrogen bond mechanism. As a result, the activation barrier for molecular oxygen dissociation is decreased leading to an increase in the surface exchange rate constant. Our study demonstrates that the SrO terminated SrTiO3 surface is not static but instead, dynamically responds to external factors such as gas composition, humidity, and temperature. The effect of the partial covalent character of cations on their ionic diffusivity in LSGM is determined computationally [3]. The diffusion of ions is assumed to take place by a vacancy-mediated transport mechanism. Using Vineyard's transition state theory, diffusion coefficients for each ion are obtained. The results of the calculations show that at temperature of 1000 K the diffusivity of the oxide ion is greater than that of the A-site cations by at least ten orders of magnitude, and the diffusivities of the A-site cations are greater then the diffusivities of B-site cations by at least eight orders of magnitude. DFT calculations were performed to elucidate the origin of catalytic activity of the pristine LnO-terminated surfaces of two Ruddlesden–Popper phase oxides of industrial interest [4]. The direct comparison of molecular oxygen interaction with La2NiO4 and Pr2NiO4 allowed us to evaluate the electronic effect on the oxygen reduction reaction energetics. We have further addressed the surface catalytic activity as a function of interstitial oxygen occupancy in the rock salt layer and provided a possible explanation for the limits of the interstitial oxygen concentration. The oxide ion transport in the rock salt layer was compared and the diffusion difference was attributed to the electronic structure of the valence shells of Pr and La. The different polarizability of those elements would lead to the opposite effect on the transition state stability. The high oxide-ion transport in three fluorite-structured oxide electrolytes is examined by using DFT [5]. Our study elucidates the oxide-ion diffusion mechanism in yttrium (Y)-doped ZrO2, Y-doped CeO2, δ-Bi2O3, and α-Bi2O3, including the major change in oxygen mobility that occurs in Bi2O3 at the δ–α phase transition. This research focuses on the partial covalent interactions, often neglected in atomistic simulations, and their effect on the migrating oxygen ion.
Westminster-O1
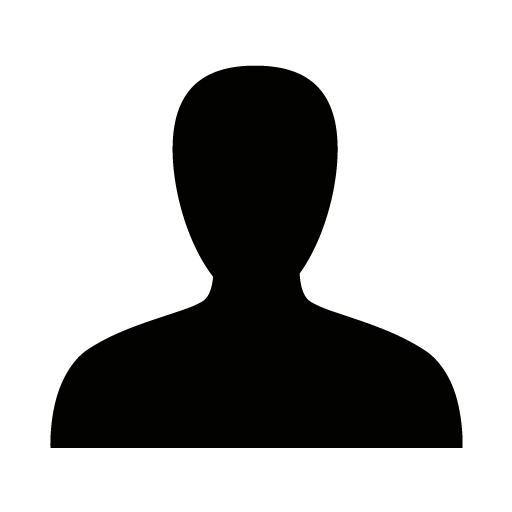
The interfacial ionic space charge effect has been used to explain various phenomena including interfacial resistance in solid-state batteries [1] and conductivity enhancement in nano-spaced superlattices [2]. Many such phenomena have later been better explained by, e.g., secondary phases [1], but in principle, there must be a space charge region to align the electrochemical potential across any interface. However, direct experimental observation of the space charge has turned out to be extremely challenging [3], and theoretical modeling has either relied on phenomenological Poisson-Boltzmann models [4,5] or first-principles calculations of interface slab models considering a limited number of defect configurations [6]. For these reasons, the atomistic picture of the interfacial space charge and its role in determining interfacial properties remain, to a large extent, unclear.
Direct first-principles modeling of the space charge is hindered by the combinatorial explosion in the number of possible defect configurations and slow relaxation times in molecular dynamics simulations. To tackle this issue using modern-day supercomputing resources, we have been developing an open-source framework abICS (for ab Initio Configuration Sampling), which combines high-throughput ab initio calculations, machine learning, and parallel statistical physics methods to enable thermodynamic sampling of millions of configurations on a lattice [7,8].
In this work, we applied abICS to the Pt/YSZ cermet interface (a prototypical high-temperature fuel cell system) to examine the segregation of dopants and oxygen vacancies at surfaces and interfaces. Comparison with calculations at varying pO2 showed that the thermodynamic conditions drastically modify the segregation behavior, with accumulation of negatively charged defects in oxidizing conditions and positive ones in reducing conditions. The space charge region was limited up to only a few atomic layers. These observations are generally in good agreement with those from Poisson-Boltzmann-type modeling [4]. From this, we may conclude that the impact of the space charge on the macroscopic properties such as ionic conductivity may be limited. On the other hand, the local structure at the surface/interface is drastically modified, implying a huge impact on the catalytic activity of oxide surfaces.
Westminster-O2
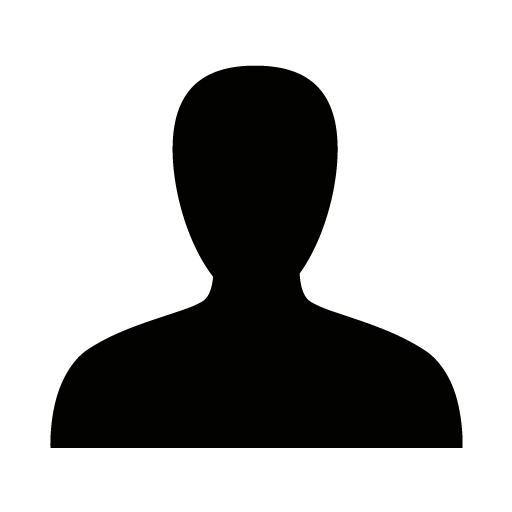
Oxide-based memory devices are an active research area due to complementary metal−oxide−semiconductor compatibility and recent dramatic increases in their performance and endurance. Many atomistic aspects of their operation are driven by the interplay between electronic and ionic processes, the mechanisms of which are still poorly understood. Recently a mechanism of electroforming process based on the formation of oxygen vacancies (VO) and interstitial O ions facilitated by electron and hole injection into the oxide has been proposed for SiO2 and HfO2 based devices [1]. Here we provide an atomistic insight into the transport of oxygen vacancies and interstitial oxygen ions, Oi, from the bulk of amorphous SiOx and TaOx, films to interfaces with TiN electrodes in the context of electroforming and performance of TiN/SiOx/TiN and TiN/TaOx/TiN devices, where x characterizes the degree of oxide reduction. In the case of SiOx, we extend the description of the bulk Oi2− migration to the interface of amorphous SiOx with the polycrystaline TiN electrode, using density functional theory (DFT) simulations. In the bulk of SiOx, interstitial migration is rapid and nondirectional in the absence of any external bias, with migration occurring in accordance with the interstitialcy mechanism [1]. In the presence of the external bias, the diffusion becomes directional, with the Oi being moved from the bulk toward the interface. At the interface, the incorporation and migration barriers for the Oi become vanishingly small, introducing a strong thermodynamic and kinetic driver for the transport of Oi to the interface. The arrival of Oi2− at the interface is accompanied by preferential oxidation of undercoordinated Ti sites at the interface, forming a Ti−O layer. [2]. We then investigate how Oi ions incorporate into a perfect and defective Σ5(012)[100] grain boundary (GB) in TiN oriented perpendicular to the interface. Our simulations demonstrate the preferential incorporation of Oi at defects within the TiN GB and their fast diffusion along a passivated grain boundary [2]. We demonstrate that in the case of TaOx, it is the hole injection that facilitates the formation of VO2+ vacancies and interstitial Oi atoms. The latter bind to Ti at the TiN interface and can be released upon bias application similarly to the SiOx/TiN case. These results explain how, as a result of bias application and carrier injection, the system undergoes very significant structural changes with the oxide being significantly reduced, interface being oxidized, and part of the oxygen leaving the system. The bias application facilitates the carrier injection into the oxide; these extra electrons or holes reduce energy barriers for the creation of O vacancies, and these barriers as well as those for O ion diffusion are further lowered by the field.
Westminster-O3
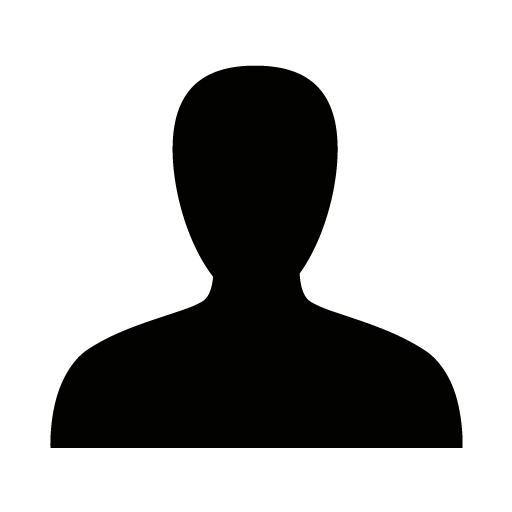
Ion intercalation, particularly with small ions, plays a pivotal role in a plethora of contemporary technologies, ranging from the realm of energy storage and conversion to physical neural networks in machine learning applications. The process involves the insertion and removal of ions into a host material without causing significant structural deformations. For example, in rechargeable metal-ion batteries, metal ions like lithium ions and sodium ions shuffle between the anode and cathode during charge and discharge cycles. Proton percolation in inorganic materials is adopted in various applications, for example, hydrogen storage, electrolyzers, fuel cells, and sensors. Similarly, proton transportation process is also essential in machine learning devices. Understanding the dynamics of ion intercalation is vital because it directly affects the performance, efficiency, and durability of systems. Typically, researchers harness the capabilities of density functional theory (DFT) and molecular dynamics (MD) simulations to attain fundamental understandings. Yet, the derived ion migration barriers and diffusivities are only average measures that inadequately elucidate complex intercalation mechanisms at the atomistic level, particularly in materials with complex structures and multiple dopants. Such approaches tend to overlook atomic dynamics as analyzing the typically vast simulation datasets remains a daunting task. Thus, while ion intercalation is undeniably significant, there remains much to uncover about its underlying intricacies.
This work focuses on understanding small ion intercalation dynamics in host microstructure at the electronic and atomistic levels, leveraging MD and advanced machine learning algorithms. We particularly focus on the proton intercalation process in scandium (Sc)-doped barium zirconate (BaZrO3) perovskite lattice, denoted as HxBZSc. MD simulations form the backbone of understanding real-time atomic interactions in our system. They provided insights by mapping the translation and reorientation jumps of protons under different operational scenarios. Given the complexity and characteristics of the trajectory data, we have applied clustering algorithms to segment the trajectory data, effectively identifying distinct patterns that highlight the dynamic interactions of protons. Additionally, visualization tools have been instrumental in deciphering these clusters, unveiling unique proton behaviors and patterns within the HxBZSc lattice models. The identified patterns indicate the existence of potential proton transfer pathways within the lattice, offering profound insights that correlate with experimental observations such as conductivity and stability. This foundational study paves the way for the development of advanced materials by enhancing our understanding of their underlying mechanisms.
St.James-K1
Storage of Lithium or Sodium in electrodes is a basic problem of Solid State Ionics and exploits point defect chemistry: Li is incorporated either as interstitial plus electron or by occupying vacancies and introducing holes.
The contribution starts with an overview of the various storage modes in battery research [1].
The simplest case is intercalation (single phase storage). The theoretical charge discharge curve is a coulometric titration curve of a mixed conductor, the practical values are governed by losses predominantly due to chemical diffusion. A master example is TiO2 [2].
The second storage mode is phase change, the classic example being FePO4. Here the stoichiometric variation around the two phases is small but most of the storage is happening in the two phase system. The defect chemistry is set out in more detail. A wonderful case is nanocrystalline FePO4 as here the two-phase region shrinks to zero and a huge stoichiometric window is met. Results from Ref. [3] are presented where it was shown that defect chemistry and only defect chemistry is able to even describe such an extreme situation.
The third mode is decomposition such as decomposition of LiRuO3 to Li2O and Ru enabling a large capacity but also showing severe kinetic difficulties. Here morphological optimization is key [4].
The fourth mode is interfacial storage [5]. The most straightforward explanation is our job-sharing model that decouples ionic and electronic effects at heterojunctions explaining excess capacities in heterogeneous systems such as Li2O:Ru. This effect also builds a bridge to supercapacitive storage. As for the latter also defect chemistry is the key, there should be a connection between interfacial storage and bulk storage, in other terms between intercalation and supercapacitive storage. Recently systematic research on TiO2 thin films has been carried out which can be translated into a unified picture of battery and supercapacitive research [6].
[1] J. Maier, Angew. Chem. Int.52, (2013) 4998.
[2] J.-Y. Shin, D. Samuelis, J. Maier, Solid State Ionics 225 (2012) 590.
[3] J. Maier, Y. Zhu, Adv. Mater. 35, (2023) 2304666 .
[4] C. Zhu, R. Usiskin, Y. Yu, J. Maier, Science 358, (2017) eaao2808 .
[5] C.-C. Chen and J. Maier, Nat. Energy 3, (2018) 102 .
[6] C. Xiao, H. Wang, P. van Aken, R. Usiskin, J. Maier, arXiv preprint arXiv:2303.10284.
St.James-I1
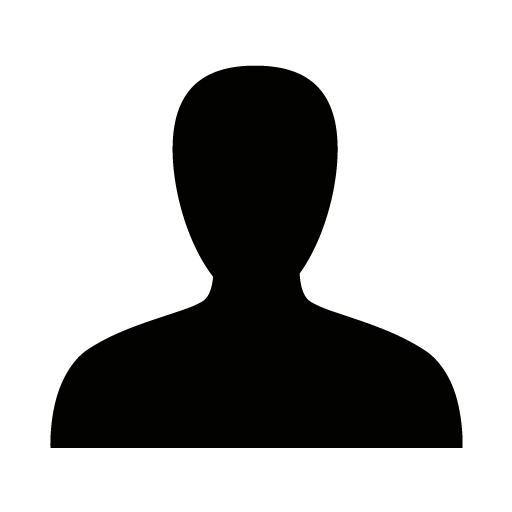
The transformation to a future renewable green economy based on new sustainable energy sources will require a new infrastructure and large amounts of new materials. The development and production of the new material has to be based on a sustainable and fair use of our resources, which demands a lifecycle in a truly circular economy. Recycling is difficult from the entropy point of view, but nearly inexhaustible solar energy can be the driver for sustainability for the foreseeable future.
The development of recyclable materials for e.g. renewable green fuel production, storage, transport and utilisation requires large-scale production from secondary raw materials. The decision making for future resilient energy systems has to be based on environmental and climate neutrality aspects as well as on performance criteria defined by a holistic life cycle assessment and thus a new dynamic metric of sustainability. The implementation of these intelligent green concepts for a future sustainable economy requires profound knowledge on environmental footprints and long-term performance factors. Such goals can be achieved by using regenerative or self-repairing materials, programmable self-disassembling and recyclable materials based on solid state ionics.
St.James-O1
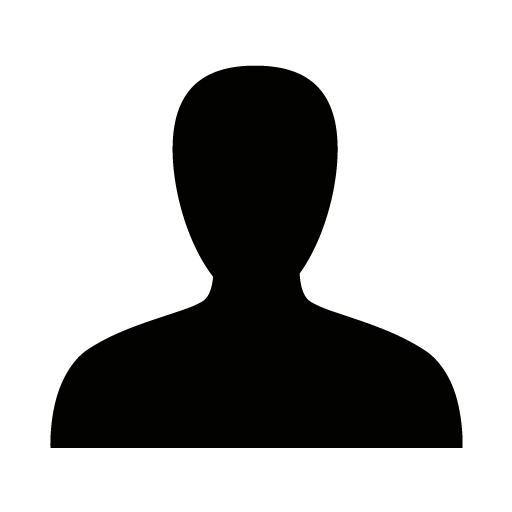
All-solid-state batteries have emerged as a potential alternative to traditional lithium-ion batteries due to their increased safety and energy density, enabled by solid electrolytes that exhibit fast ion conduction. While many new ion-conducting solids have been reported recently, further improvements in ionic conductivity are needed to achieve practical cell configurations such as a thick cathode design. In addition to the long-studied sulfide and oxide electrolytes, Li-ion conducting halide-based SEs have recently attracted enormous attention owing to their electrochemical, mechanical, and transport properties, compromising those of oxides and sulfides. Despite the success of Li-ion conducting halides, the research on Na-analogue remains sparse, and the reported ionic conductivities are limited. In this study, we demonstrate the significantly improved ionic conductivity in NaM5+Cl6 (M5+ = Nb, Ta) by extending the existing structural framework of the Na-ion conducting halides Na3M3+Cl6 (M3+ = Y, Er).
NaM5+Cl6, crystalizing into the monoclinic structure with the space group of P21/n, possesses close structural relationships with Na3M3+Cl6 (space group: P21/n) and Na2M4+Cl6 (space group: P21/n). The structure of Na3M3+Cl6 (space group: P21/n) is a heavily distorted double-perovskite structure (A2BB’C6: A = Na1, B = Na2, B’ = M3+, C = Cl) with two Na positions. With increasing the content of M4+ cations, the Na2 site becomes vacant to compensate for the charge, and the structure becomes unstable. However, a further increase in the valency of M elements to M5+, e.g., NaNbCl6 and NaTaCl6, stabilizes the structural framework of the monoclinic phase of Na3M3+Cl6 and Na2ZrCl6 again, at room temperature. Both NaNbCl6 and NaTaCl6 form a monoclinic structure with the space group of P21/n with alternating Na and vacant layers along the c-axis, and, as a result, the structural cation of M5+ is displaced from the center of the octahedra to take distance from the Na layers.
In this study, we successfully synthesized the two series of solid solutions Na1+xNb1−xZrxCl6 and Na1+xTa1−xZrxCl6 and investigated their transport properties. In both series, the ionic conductivity peaks at around x = 0.2, likely due to the enhancement in the migration entropy. However, the achievable conductivity with Ta is an order of magnitude higher due to the lower activation energy despite the almost identical unit cell sizes. We will discuss the possible reasons for this difference in the activation energy to extend it to the general design principles.
St.James-O2
Concept of Na-ion batteries is among the most promising battery technologies to deal with the issue of critical and scarce elements used in Li-ion batteries. Portable applications demand high energy density, favoring V- and Mn-based Na-ion positive electrode materials. In contrast, for stationary applications where battery weight is less critical, component cost becomes a more compelling factor. Stable polyanionic frameworks ensure longer cycling life as well as greater safety in comparison to layered oxides. In 2023 Mn has been classified as a critical raw material by EU committee, which promotes search and development of new materials based on abundant Fe. For the polyanionic part, phosphates are widely studied, leaving space for investigation of pure sulphate phases and in parallel for mixed phosphate-sulphates.
From this point of view, we revisited already reported NaFe2PO4(SO4)2 (NFPS), which crystallizes in NaSICON structural type [1-4]. High-purity samples of NFPS were synthesized and studied by means of X-ray, neutron and electron diffractions, scanning electron microscopy and electrochemical methods. A new description of the structure was proposed and the relationship between synthesis conditions, stoichiometry, structure, and electrochemical performance will be discussed. Optimization of morphology and electrode formulation allowed us to obtain theoretical capacity at C/30 cycling rate (mass loading 20 mg/cm-2). Step-by-step up-scaling led to synthesis of 8 g of NFPS per batch.
Attempts to synthesize sodiated compound of the same element compositions as NFPS via easy up-scalable solid-state synthesis result in new alluaudite-type series of phases. Challenges of the synthesis linked to control of stoichiometry and promising electrochemical performance will be presented.
A new phase based on only abundant elements, such as Na, Fe and S, obtained via simple ball-milling procedure, could be suggested as a cheap and efficient positive electrode material for stationary storage. Its synthesis requires only two easily-available precursors and no heating is required. One of the main advantages of this material is excellent stability of cycling. Structure and electrochemical experiments would also be presented.
St.James-O3
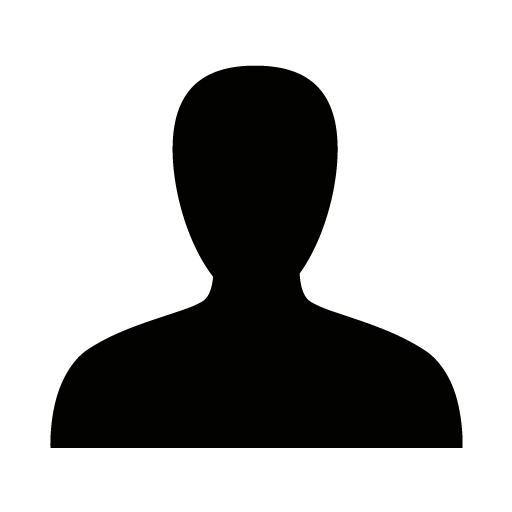
Solid-state sodium batteries (Na-SSBs) are emerging as a promising technology due to the abundance and low cost of sodium, offering an attractive alternative to lithium batteries with similar electrochemical properties. This study marks a breakthrough in solid-state sodium batteries by focusing on “anode-free” or “reservoir-free” Na-SSBs. Unlike traditional batteries, this approach eliminates the to include the metallic negative electrode during assembly. Instead, the cell is assembled with an "empty" negative electrode, dynamically formed during the initial charge cycle. This will enhance the safety and simplicity of the assembly process by removing the most reactive component of the cell, providing a high purity in-situ formed metallic anode with higher practical energy density(no excess Na added). The Na3.4Zr2Si2.4P0.6O12 (NZPS) NaSICON has numerous advantages such as an increased safety, greater stability, and higher energy density. In addition, NZSP has a remarkable ionic conductivity (up to 5*10-3 Scm-1)1,2 and a high resistance to moisture and air. It has recently been recognised to facilitate rapid charge transfer at the Na metal/NaSICON interface by stabilising a conductive solid-electrolyte interface (SEI) layer through thermally activated surface decoration.
Understanding and optimising the interface between NZSP and sodium is crucial, as it is indispensable to prevent the formation of an SEI layer that could negatively affect the cell performance. Furthermore, investigating sodium nucleation in anode-free batteries, where the anode is formed in situ, is essential to understand its interaction with the electrolyte-current collector interface. Several factors come into play, such as the chemical and morphological nature of the interface and the interaction of Na the system during platting and stripping.
The choice of current collector is paramount as it interacts with the electrolyte surface and can play a pivotal role on the nucleation overpotential and stability of the interface upon cycling. By altering the morphology, size and composition of the current collector, we can investigate the growth of sodium and its interaction with both the electrolyte surface and the current collector. For example, metals including alloying (Sn) and non-alloying (Au) are examined for their respective effects on battery performance. Important factors include variations in nucleation energy to facilitate reversible cycling and stability. Understanding these aspects is crucial to optimize battery performance during the charging process.
Electrochemical experiments have been conducted to study the interface, employing both symmetric cells and anode-free cells with various electrolyte surfaces and current collectors. Additionally, modified surfaces have been analyzed using characterization techniques such as XPS and Raman spectroscopy.
Moore-K1
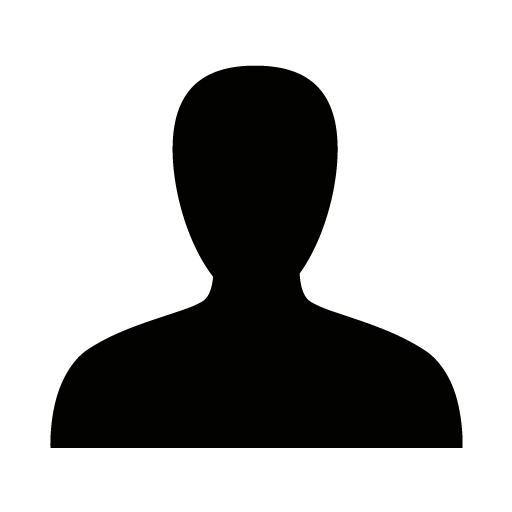
Point defects are a universal feature of crystals. Their identification is addressed by combining experimental measurements with theoretical models. The standard modelling approach is, however, prone to missing the ground state atomic configurations associated with energy-lowering reconstructions from the idealised crystallographic environment. Missed ground states compromise the accuracy of calculated properties. To address this issue, we report an approach to navigate the
defect configurational landscape using targeted bond distortions and rattling. Application of our workflow to eight materials (CdTe, GaAs, Sb2S3, Sb2Se3, CeO2, In2O3, ZnO, anatase-TiO2) reveals symmetry breaking in each host crystal that is
not found via conventional local minimisation techniques. We then explain the point defect distortions are classified by the associated physico-chemical factors. Lastly, we illustrate how important this analysis is on a database of oxygen vacancies in
>300 oxides. To conclude, we propose guidlines for best practice in reporting defect chemistry/physics results to ensure transparency and reproducability.
Moore-I1
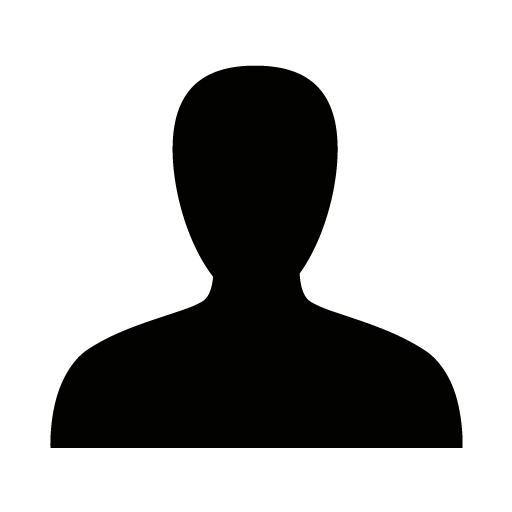
Materials engineering through thin-film nanostructuring represents an ideal playground for the investigation of model systems with novel or improved functionalities. In the context of high-temperature cathode materials, improved kinetics has been achieved, notably through strategies such as grain boundary engineering and heterostructuring.1,2,3 Despite these advancements, however, an open question pertains to the practical relevance of such structures in real electrochemical devices. Existing constrains such as processability and long-term thermochemical stability have considerably hindered, to date, the application of advanced thin-film technology to high-temperature energy devices like solid oxide cells. This contribution addresses these challenges by presenting practical examples that demonstrate how the strategic introduction of defects can be leveraged in order to optimize the performance-stability trade-off in electrochemically active films for oxygen reduction reaction. For 0-dimensional defects, we will introduce results on a combinatorial study for the (La,Sr)(Mn,Fe,Co)O3 system. Employing a suite of automated and semi-automated characterization tools, we were able to map the electrochemical behavior of the ternary compositional space, demonstrating that aliovalent cationic substitution is an effective tool for balancing high electrochemical activity and enhanced thermal stability. Another example showcases our latest findings on perovskite/fluorite self-assembled nanostructures.4 Here depending on the materials of choice, distinct long-range orders are obtained. Despite the architecture variations, a general increase in the oxygen-reduction kinetics (over one order of magnitude) is achieved with respect to single-phase reference materials. Furthermore, the long-term stability of the material operating under real conditions is demonstrated. Finally, the successful implementation of such thin-film systems as high-performance cathodes in full solid-oxide cells is shown, highlighting the potential of thin-film engineering for practical implementation.
Moore-O1
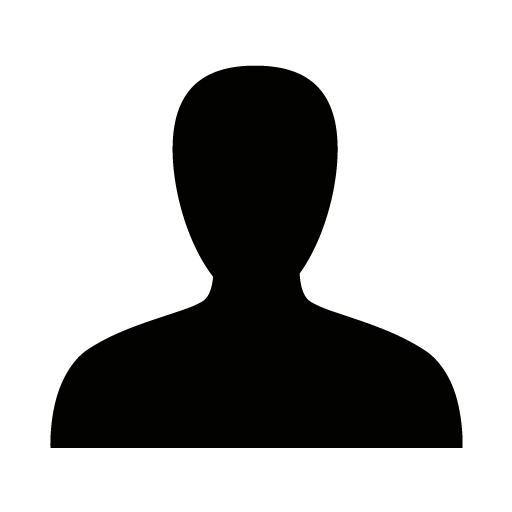
Low temperature micro solid oxide cells (µSOCs) represent a key technology in next-generation energy devices for portable applications, offering the highly efficient conversion of electrical to chemical energy (e.g. hydrogen), and vice versa. To date, the widespread implementation of µSOCs, and therefore the effective use of hydrogen as a portable energy storage solution, has been prohibited by excessive polarisation resistances at the device electrodes, despite significant progress in materials design.
Vertically aligned nanocomposite (VAN) films have been a leading class of materials in recent performance enhancements in µSOC design, and in this work we further explore the growth and characterisation of such VAN films. State-of-the-art fluorite and perovskite materials are combined in unique nanocomposite structures and the enhancement rendered with respect to equivalent planar films is quantified by electrochemical impedance spectroscopy (EIS). Films are grown on both single-crystal and more commercially viable polycrystalline/amorphous substrates. This allows for a detailed study of the VAN growth mechanisms for materials of differing crystal structures, while also giving an improved understanding of the importance of crystalline perfection in thin-film µSOC device performance.
Future progress in low-temperature µSOC technology will rely heavily on a detailed understanding of the mechanisms underpinning the performance enhancements achieved in such nanocomposite thin films. Therefore, by building an understanding of the growth mechanisms of state-of-the-art nanostructured materials and quantifying the performance enhancements resulting from a wide variety of VAN structures, this study represents an important step towards the realisation of efficient low-temperature µSOCs for portable applications.
Moore-O2
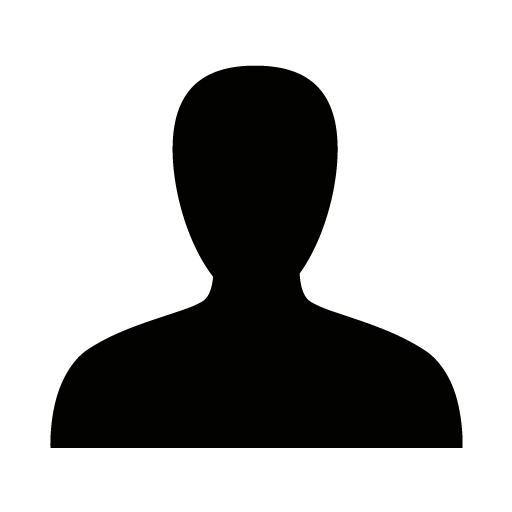
We present a universally applicable measurement technique for studying diffusion in thin films. By covering parts of the measured sample with a protective layer, the electrochemical insertion of ions into the thin films can be locally confined to a small stripe-like gap, allowing us to explore lateral diffusion of inserted species away from the gap into the thin film under the protective layer. By using this method, it is possible to perform in situ Raman measurements at various distances from the gap. This allows us to record temporally and spatially resolved information about the crystal structure and optical properties of the sample. Raman measurements at a fixed position allow us to probe changes in crystal structure as the concentration of the inserted species varies. As the distance from the gap increases, the diffusion profile becomes more extended and the concentration gradients become smaller. Therefore, the temporal resolution of the measurements improves significantly. When combined with spatially resolved transmission measurements, this approach can provide detailed information about the crystal structure and diffusion profile of the thin films under investigation.
We used this method to investigate tungsten trioxide thin films and their electrochemical behavior with respect to hydrogen-induced structural phase transitions and their impact on the diffusion process. Spatially resolved transmission measurements in the wavelength range of 633±55 nm provide the diffusion profile perpendicular to the stripe like gap and are used to characterize the diffusion. The time-resolved Raman measurements at different distances from the electrolyte contact area are conducted with a 633 nm laser. Our results reveal an unambiguous correlation between the structural phase transition of the thin film (from the orthorhombic to the tetragonal phase) and the variation of the diffusion coefficient with hydrogen content. A larger diffusion coefficient of the tetragonal phase compared to the orthorhombic phase is due to the higher symmetry of the latter.
Furthermore, a numerical simulation was conducted whose results support the findings regarding the concentration-dependent diffusion.
Abbey-K1
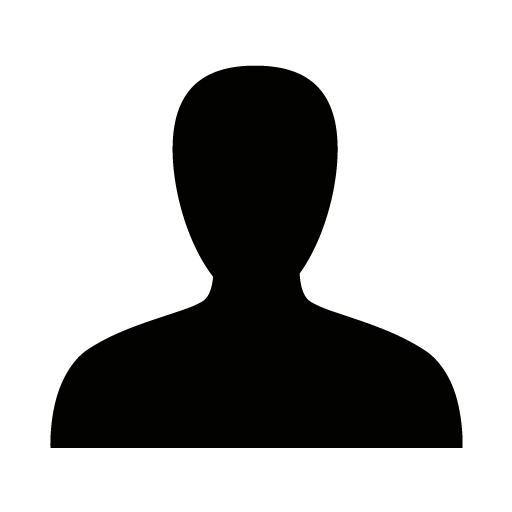
This talk will describe how a combination of NMR to measure transport, X-ray diffraction to measure phase segregation within the bulk, optical measurements and electron microscopy to explore processes at the particle level, and continuum modeling to tie transport with particle level observations, can be used to study electrode material function and failure. First, NMR measurements provide hopping frequencies and diffusion coefficients. Second, operando diffraction measurements provide evidence for solid solution vs two phase behaviour as a function of cycling. New optical measurements allow phase transformation and phase fronts to be visualized directly. The processes that underpin the various phase fronts can then be explored via simulations. This is illustrated with nickel rich layered cathode materials such as NMC-811. The approach is extended to study the end member of this series LiNiO2 where the effect of phase transitions at the top of charge (i.e., when most of the Li is removed), has significant implications for particle degradation via processes such as cracking (along with oxygen loss). Finally, the role that transport, grain boundaries and surface impurities have on dendrite formation in oxide-based solid-state battery electrolytes will be discussed.
Abbey-I1
Rechargeable batteries can contribute to powering electric transportation and storing electrical energy generated from intermittent renewable sources. Li ion batteries (LIBs) are the current battery of choice and new types of batteries such as solid-state Li metal batteries (SSLMBs) are also under active research. There are constant demands for further improving rate capability and energy density of batteries. Thick electrodes can increase energy density at the cell level, but one of the limiting factors is slow ion transport kinetics that reduce capacity at increasing (dis)charge rates. This problem is exacerbated in SSLMBs as the ion transport coefficient is usually lower in solids than in liquids. Here, two novel processing technologies have been developed to optimise the battery electrode microstructure and improve ion diffusion kinetics: (i) directional ice templating (DIT) for fabricating thick (900 µm) cathodes with vertical pore arrays and porosity gradient for LIBs [1]; and (ii) directional freezing and polymerisation (DFP) for fabricating cathodes with vertical arrays of solid polymer electrolyte (SPE) directly incorporated in the cathode microstructure during processing for SSLMBs [2]. Both techniques reduced tortuosity τ of ion diffusion pathways through electrode thickness from ~3.3 for conventional electrodes to 1.5.
A new operando correlative imaging technique of combining X-ray Compton scattering imaging (XCS-I) and computed tomography (XCT) has also been developed that allows pixel-by-pixel mapping of Li+ chemical stoichiometry variations in a LiNi0.8Mn0.1Co0.1O2 composite cathode within a coin cell battery [3,4]. This technique shows how the anisotropic electrode microstructure improved Li+ ion diffusivity, homogenised Li+ ion concentrations, and improved energy storage performance.
Abbey-O1
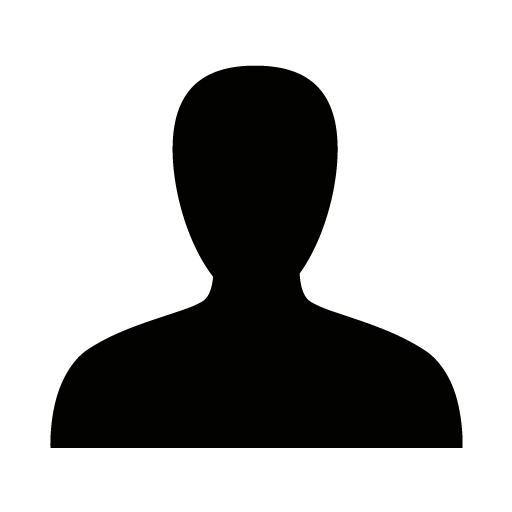
For battery development there is a need to produce structured component electrode for optimised electrochemical and recycling performance.
3D printing is an ideal method to approach this challenge as it allows controlled deposition of materials in an interconnected manner.
The introduction of 3D extruded electrodes enables quality-oriented manufacturing, by reducing environmental impact and design for recycling- oriented approach.
In the standard electrode format high tortuosity mitigates Li-ion diffusion which results in poor performance at high discharge rates. Moreover, mixed two-component electrodes, comprising of multiple components make efficient recycling of active materials challenging.
The most optimal way to achieve 3D structured high energy electrodes produced in a high through output manner is to concurrent 3D printing of multi-material components and multi-nozzle set up. Multi-material 3D printing enables reduction of the deposition time making the process controllable and time efficient.
In this research, negative electrodes of two chemistries: graphite and hard carbon together with SiOx have been investigated. The designed 3D structured electrodes, printed via the Direct Ink Writing (DIW) extrusion process, were aimed to overcome difficulties associated with traditionally doctor-blade or slot-die coated negative electrodes.
The electrode design was targeted at direct-loop recycling that aims to recover the active materials in separate streams in order to maintain the original properties of the active materials and to eliminate cross-contamination during recycling process. As it is industrially important to recover electrode constituents as two separate streams when the battery reaches an end-of-life to achieve efficient recycling and enable re-manufacturing.
In this work we introduce twofold property of the channels: low tortuosity electrodes result in enhanced Li-ion diffusion. Power and energy density were improved by uniform distribution of active material and conductive additives. Improved separation and recovery efficiency of components were achieved by controlled wettability of the DIW printed electrodes.
Abbey-O2
Li metal solid state batteries (SSBs) enable high energy and power density and improve the overall safety of the device over conventional LiBs [1]. Removing the lithium metal anode solve critical problems ascribed to SSB manufacturing and increase the final energy density by optimizing the cell volume. In an anode-less battery, the Li-metal plates and strips on the bare current collector during cycling. The feasibility of this configuration in a SSB has already been demonstrated in several publications, although low coulombic efficiency and deeper understanding of interfaces are major challenges [2], [3], [4].
The aim here is to demonstrate the interfacial optimization of anode-less solid-state battery with Li7La3Zr2O12 (LLZO) electrolyte. Anode-less cells were assembled with various sputtered current collectors (CC) such as Cu and Ag. Cells with various Cu CC thicknesses were cycled to analyze electrodeposited Li morphology by post-mortem SEM analysis. Current collector and electrolyte surfaces were analyzed by Atomic force microscopy (AFM), Raman spectroscopy and Scanning electron microscopy (SEM), before and after cells cycling. Li nucleation and growth were further studied by Galvanostatic impedance spectroscopy (GEIS) to understand the complex nature of physical/chemical phenomena during in-situ plating of Li.
We found that Li nucleation potential is independent of CC thickness, however electrodeposited Li showed different morphology depending upon CC thickness. For the first time cells with Cu current collector and LLZO electrolyte worked up-to 100 cycles with a Cu layer as thin as 50nm and at very low nucleation potential of ~30mV. By addition of nanometric Ag interlayer in between LLZO and Cu, we could effectively control Li deposition [5]. The robust thin film interlayer enabled stable cycling, accommodating the volume changes without the need for extra external pressure.
Abbey-O3
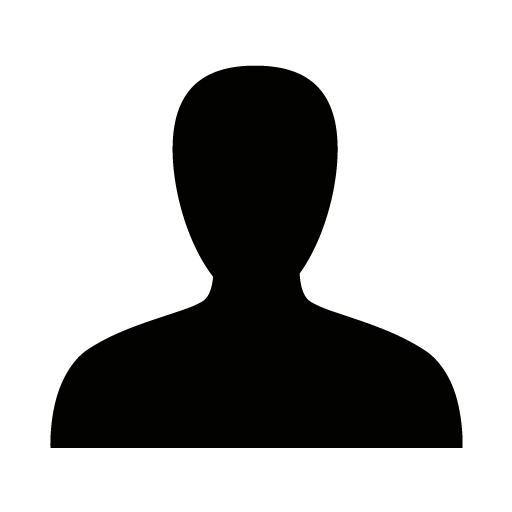
Lithium-ion batteries (LIBs) are one of the most promising energy devices owing to their high power density and long-term stability. In particular, the graphite anodes have been predominantly used as the negative electrode due to the good cycling stability, electrolyte compatibility and low cost. However, graphite anode degradations have a significant impact on the overall battery capacity during battery operations. Notably, solid electrolyte interphase (SEI) formation and volume expansion known as the most common anode degradations are challenging to detect through the experiments, thus there has been a growing trend in using computational simulations for anode degradation research. However, most of the research related to the identification of graphite anode degradation mechanisms by the simulations have not considered the chemical and mechanical degradations at the same time during the battery operation process. Also, they underestimate the heterogeneous effect of the anode electrodes. Therefore, we present an electro-chemo-mechanical 4D-resolved model for anode degradation during various charging rates using the heterogeneous graphite anode structures. From this work, we identify a realistic representation of the correlation between chemical and mechanical anode degradations. Our model provides a comprehensive understanding of the complex anode degradation mechanisms.
Gielgud-K1
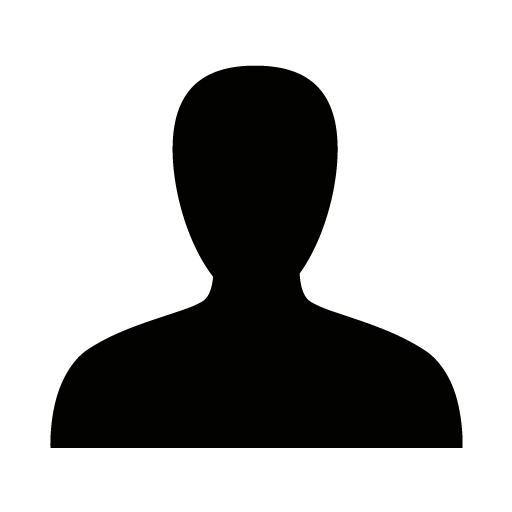
The mandatory need of an energy sources diversification leads to a current great interest for the research areas related to hydrogen technologies. In particular, with a high efficiency Solid Oxide Cells produce either hydrogen when used in the electrolysis mode (Solid Oxide Electrolysis Cell, SOEC) or electricity and heat when used in the Fuel Cell mode (Solid Oxide Fuel Cell, SOFC). However, the durability in operation (especially in the SOEC mode) needs to be improved by reaching less than 1% degradation per 1000 h. To reach this objective, a solution is to decrease the working temperature (i.e. down to 600°C), without decreasing the power density. Approaching these objectives is really challenging.
For this purpose, ICMCB-CNRS follows several routes from long time in close collaboration with partners belonging to the French Research Federation on hydrogen (FRH2, https://frh2.cnrs.fr/), CEA and industrial partners. The French program of research PEPR-H2 (https://www.pepr-hydrogen.com/) leaded by CNRS and CEA is a favorite frame.
In this presentation, I will detail the most recent developments performed at ICMCB in collaboration with IRCER-CNRS laboratory and CEA-Liten, following three main axis. The first one concerns the research of new materials of electrodes, mainly oxygen electrodes, recently we had focused on some materials belonging to the upper terms (n=2, 3) of the series Lnn+1NinO3n+1±d (Ln = La, Pr), as well as doping on the anionic site (oxygen) by fluorine, for instance regarding reference electrodes such as LSFC (La-Sr-Fe-Co). The second axis concerns the development of innovative shaping routes of such multi-layered cells. A first goal was to produce a Solid Oxide Cell entirely composed of Gd-doped ceria with functionalized (impregnated) porous electrodes. The second goal was to develop a simple and low cost shaping process, i.e. the tape casting. Then we have developed a combined tape casting and infiltration (of solution) method in order to prepare new architectures of SOC(s). Finally, to bear our research we have developed several measurements tools, such as for instance IEDP (Isotopic Exchange Depth Profiling) measurements performed under oxygen pressure, i.e. close to the usual conditions of the SOEC devices.
Gielgud-I1
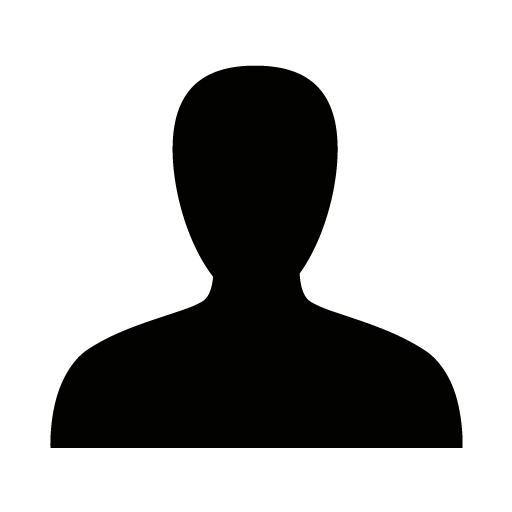
Proton-conducting solid oxide electrolytes are highly desirable for steam electrolysis (PCEC) and fuel cells (PCFC) application at moderate to low temperatures, given their high ionic conductivity and inherent advantages in the gas flow configuration over traditional solid oxide cells in which the electrolyte is an oxygen ion. Despite the progress made with small-scale laboratory cells in recent years, a significant challenge continues to be upscaling robust and affordable planar-type devices. This paper presents recent updates on our effort toward scaling PCEC/PCFC devices using an industrially established inverse tape-casting route. Our cells are constructed using NiO-SrZr0.5Ce0.4Y0.1O3-δ as the substrate, which ensures minimal warping and no cracks in the end-fired state. After co-sintering at 1300 °C for 5 hours, the tri-layered green tapes achieved dense, gas-tight electrolyte layers with a He leakage rate well within the threshold necessary for cell operation (~5 × 10–5 hPa dm3 (s cm2)–1). Using Ba0.5La0.5CoO3−δ as the air electrode demonstrates remarkable capabilities and endurance within the 450-600°C temperature range, achieving a remarkable peak power density of 1.1 W cm-2 at 600 oC in the fuel cell mode and a high current density of 1.5 A cm-2 at 1.3 V in the electrolysis mode. Furthermore, scanning electron microscopy in combination with energy-dispersive X-ray spectroscopy, Raman spectroscopy, and Atom probe tomography (APT) were used to analyze the structural modifications of the half-cells upon sintering at varying temperatures. It is apparent from the latter techniques that upon sintering above 1350 °C, the electrolyte material undergoes evident structural changes with new crystal defects that affect the perovskite host. The APT analysis clarifies the distribution and concentration of segregated cations around the grains' vicinity. These issues and polarization processes that influence performance in the fuel cell mode identified through impedance spectra analysis will be presented.
Gielgud-O1
The development of highly efficient and flexible technologies based on renewable energy resources are essential for the industry decarbonization. In the case of pure oxygen production, oxygen transport membranes (OTMs) appear as an alternative technology for the cryogenic distillation of air, the most common technology for oxygen production. This kind of membranes are controlled for his mix ionic and electronic conductivity (MIEC) and a difference of pO2 between both chambers. In addition, OTMs could provide oxygen from different sources (air, water, CO2, etc.), and they are more flexible to adapt to current processes, producing oxygen at 700-1000 °C. In this matter, decrease the reactor temperature could effect in the global efficiency, improving the operability of this membranes in different processes.
The first part of this study focuses on electrification on a traditional OTM material (Ba0.5Sr0.5Co0.8Fe0.2O3-d), imposing different electric currents/voltages along a capillary membrane. Thanks to the emerging Joule effect, the membrane-surface temperature, and the associated O2 permeation flux can be adjusted. Here, the OTM is electrically and locally heated and reaches 900 °C on the surface, whereas the surrounding of the membrane was maintained at 650 °C. The O2 permeation flux reached for the electrified membranes was ~3.7 mL/min·cm2, corresponding to the flux obtained with an OTM non-electrified at 900 °C. Furthermore, OTMs can be integrated in catalytic membrane reactors, providing new pathways for different processes, like oxidative dehydrogenation, partial oxidation, etc. In this study was evaluated the effect of the electrification in oxidative dehydrogenation of ethane (ODHE), taking to account that the ODHE reaction is very sensitive with the temperature. Our results indicate that OTM electrification improves the ODHE reaction by achieving higher yields to ethylene if compared to the non-electrified membrane, viz. 40% and 10%, respectively. In summary, this work highlights the benefits of membrane electrification by decreasing the operational temperature, which can be applied to several reactions for fuel and chemicals production enabling higher conversions and selectivity to targeted products.
Gielgud-O2
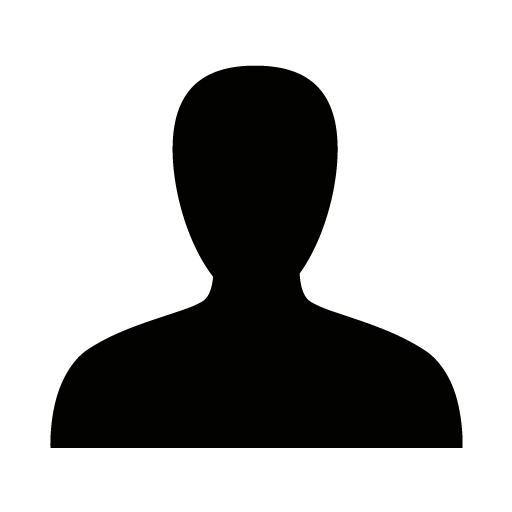
Chemical looping for hydrogen production (CLHP) shows the potential to substantially lower the CO2 emissions from H2 production with hydrocarbon sources [1]. In CLHP, an oxygen-carrying material (OCM) is reduced by a hydrocarbon source to produce syngas, followed by partly oxidation with steam to generate pure H2, before re-oxidation with air to balance the energy demand [2]. However, commercialization of CLHP is still limited by the OCM which should possess a demanding set of properties; catalytic activity towards the red-ox processes without forming coke, sufficient oxygen transport and storage capacity, chemical and microstructural stability over numerous redox cycles, and be abundant with no environmental and societal issues [3].
Ca/Sr1-xLaxBO3 (B = Ti, Cr, Mn, Co, or Fe) perovskites are interesting OCM candidates, showing good chemical stability, high mixed ionic- and electronic conductivity and reversible oxygen stoichiometry over a wide range of reaction conditions[4-6]. In this work, a matrix of Ca0.6La0.4Ti0.1CrxMn0.9-xO3-δ, x = 0, 0.3, 0.45 and 0.9, has been studied to investigate the effects of the Cr/Mn content on oxygen exchange kinetic, chemical stability and oxygen stoichiometry.
Pulse Isotope Exchange measurement was utilized to investigate the oxygen exchange of the different compositions as a function of pO2 and temperatures to determine the total oxygen exchange rate (ℜ0), as well as, rates for dissociative adsorption (ℜa) and incorporation (ℜi). Chemical stability and oxygen stoichiometry of the different compositions were tested by thermogravimetric analysis under simulated CLHP operating conditions, followed by XRD and SEM.
These novel materials show excellent chemical stability and adequate regenerative oxygen stoichiometry over the whole CLHP range, of which Ca0.6La0.4Ti0.1Cr0.3Mn0.6O3-δ exhibits the best overall performance. Variations in the oxygen exchange kinetics and capacity, and the chemical stability, will be discussed with basis in the material’s point defect thermodynamics.
Fleming-T1
Discovery of fast proton conductors can significantly advance a wide range of technologies, including hydrogen fuel cells, electrolyzers, electrosynthesis of fuels, batteries as well as brain-inspired computing devices. Especially the latter needs inorganic, fast proton conductors at room temperature. A quantitative understanding of the physical traits of a material that regulate proton conduction is necessary for accelerating the discovery of fast proton conductors. While electronic and structural descriptors have been found to facilitate proton conductivity, the role of lattice dynamics remain unexplored quantitatively, albeit hypothesized to be important in affecting proton conduction.
In this work, we have mapped the structural, chemical and dynamic properties of solid acids and ternary oxides to the elementary steps of the Grotthuss mechanism of proton diffusion. Our approach combines ab initio molecular dynamics simulations, analysis of phonon spectra and atomic structure calculations.
In solid acids, we have identified the donor–hydrogen bond lengths and the acidity of polyanion groups as key descriptors of local proton transfer, and the vibrational frequencies of the cation framework as the key descriptor of lattice flexibility. The latter facilitates rotations of polyanion groups and long-range proton migration in solid acid proton conductors. The calculated lattice flexibility also correlates with the experimentally reported superprotonic transition temperatures. Using these descriptors, we have identified potential solid acid proton conductors that go beyond the traditionally considered chemistries.
For ternary oxides, the oxygen sublattice presents the donor and acceptor sites for proton conduction. While the metal-oxygen polyhedra are not nearly as flexible to rotate as in solid acids, the thermal fluctuations of O…O distances is a good measure of the flexibility of this class of conductors. We hypothesize that phonon modes which induce large fluctuations in O…O distance and shorten H…O bond lengths can assist with proton hopping. Supporting this hypothesis, we found correlations between the thermal O…O fluctuations and calculated proton hopping barriers for 10 ternary oxides with varied crystal structures. These results imply that both static O…O distance and dynamic fluctuations must be considered to predict proton conductivity in ternary oxides.
These physical descriptors of proton conduction also provide paths for increasing the conductivity at low temperature. With the rapid growth of material databases, our approach lays ground for physically informed search of fast proton conductors and enlarges the chemical space of materials to power the green revolution.
Fleming-K1
PhD : "New bi-dimensional oxide ion conductors of Aurivillius type ", 1992, supervised by Professor Gaëtan Mairesse, Laboratoire de Cristallochimie et Physicochimie du Solide, UMR CNRS 8012.
HDR : "Oxide ion conductors with low dimensionality - Correlation structure-properties", Lille, 1999.
2000-2001 : Marie Curie fellowship at Imperial College London in the group of Professor John Kilner, « Characterisation of oxygen transport phenomena in bismuth based materials by isotope exchange of oxygen ».
Head of the Ecole Nationale Supérieure de Chimie de Lille since 2016.
Researcher at the Unit of Catalysis of Solid State Chemistry in the field of Solid Oxide Fuel Cell.
Research and characterisation of new materials for use as air electrode in SOFC oe SOEC.
In contrast to main solid oxide cell air electrodes whose structures derived from the perovskite, Ca3Co4O9+d (CCO) and Ba2Co9O14 (BCO) are built upon CoO2 layers of CdI2 type. Despite they low oxide ion conductivity, there are currently considered as promising solid oxide cell electrodes not only for Solid Oxide cells but also for Protonic Ceramic Cells. In composite with Ce0.9Gd0.1O1.95 (CGO), an Area Specific Resistance of only 0.08 ohm.cm2 was obtained at 700°C on a symmetric cell made of 50% of BCO and 50% of CGO [1]. These high performances were recently confirmed by Araùjo et al who reported an ASR of only 0.3 ohm.cm2 at 600°C for a composite with Ce0.8Gd0.2O1.9 [2]. In case of CCO, the ASR at 700°C was decrease from 4 ohm.cm2 at 700°C for the pure compound prepared by solid state route to 0.35 ohm.cm2 when combined with CGO after optimisation of the microstructure and electrode thickness [3]. The performances were even increased when a strontium doped composition was used, reaching 0.15 W.cm2 for the ASR at 700°C. Thanks to a thermal expansion coefficient for CCO in the same order of magnitude than those of the electrolytes currently used for the application, a very good electrolyte/electrode interface was noticed after full cell test, making this material promising not only in terms of performances but also durability. Here, the state of the art of these two promising electrodes will be presented.
Fleming-I1
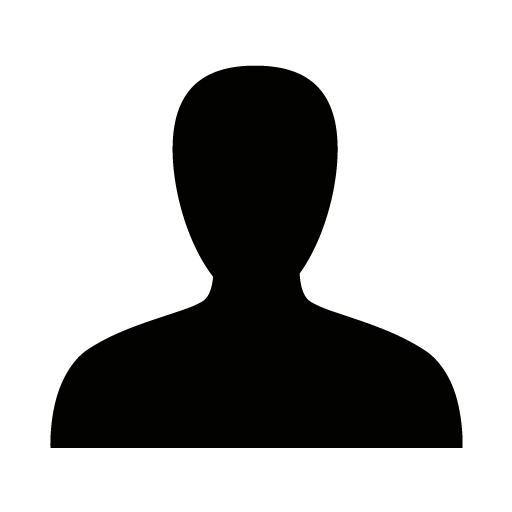
The surface exchange reaction is, while technologically relevant, poorly described for many mixed ionic-electronic conductors (MIECs). Due to the complex, multi-step nature of the exchange reaction, over the years there have been efforts to develop empirical correlations between the exchange reaction and properties that are easier to measure, calculate, or engineer. These include, concentrations of defects, diffusion coefficients, and the position of the conduction band, valance band or Fermi level. All suffer from the same limitations: they are based on bulk properties.
Given that the oxygen exchange reaction takes place on the outer surface, it is not surprising that the surface chemistry plays a significant role in the oxygen exchange rate. Intuitively, one might expect increases in the exchange rate to come from the addition of redox-active transition metal species, however, recently Nicollet et al. demonstrated that the surface exchange rate of Pr0.1Ce0.9O2 (PCO) could be altered by more than 5 orders of magnitude by the infiltration of nominally inert, isovalent binary oxides, and that the change in the exchange rate is correlated with the acidity of the infiltrated species.[1] Experimental work from Riedl et al. have confirmed these findings,[2] and an increasing body of work have shown that this correlation extends to multiple MIEC systems.
Attempts to provide a mechanistic explanation have primarily focused on the role of electron transfer between the modified surface and adsorbed oxygen species. In this work, based on experimental data from model MEICs systems and an analysis of literature, we present an alternative hypothesis, which considers, instead, the role of water species from the gas atmosphere.
Fleming-O1
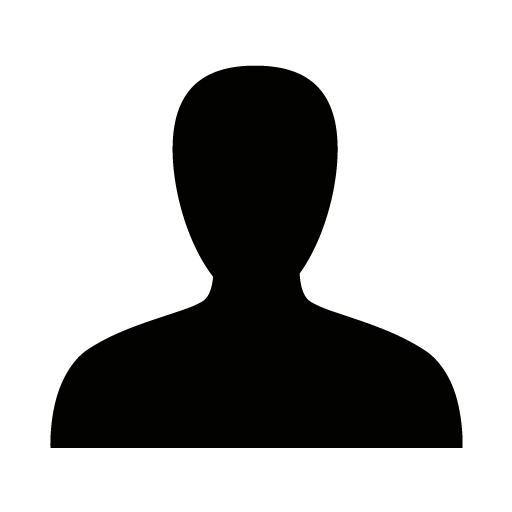
Understanding oxygen exchange kinetics at the surface of solid oxides is crucial for different applications such as fuel cells and sensors… It is known that small amounts of impurities at the surface of mixed conducting oxides can dramatically affect oxygen exchange kinetics. In a recent study [1], it has been showed that the effect of an impurity oxide at the surface of a mixed ionic and electronic conducting oxide (MIEC) can be directly linked to their acidity. Following an acidity scale proposed by Smith [2], it has been showed that basic impurities tend to enhance oxygen surface exchange kinetics, while acidic impurities tend to decrease it. However, this previous study only considered single valence oxides as impurities, to avoid competitive effect of potential redox/catalytic properties of the impurity oxide. This means that the effect of transition metal oxides impurities remains to be determined.
In this work, we studied the effect of the infiltration of transition metal oxides on O2/MIEC surface exchange and adsorption properties. Pr-doped ceria (Pr0.1Ce0.9O2-δ) was used as a host mixed conducting oxide, and a wide range of transition metals oxides were infiltrated on Pr-doped ceria samples to study their effect on oxygen surface exchange kinetics by conductivity relaxation. Variation of reaction rates is discussed with respect to chemical properties of the transition metal oxides, including their acidity (Smith scale), their crystal structure, and redox properties. Adsorption properties were also studied by conductivity measurement coupled to gas phase analysis by mass spectrometry. Adsorption properties are then linked to kinetic performances.
Westminster-K1
Prof. Dr. Regina Dittmann received a degree in Physics from the University of Cologne, Germany in 1990 and the PhD in Physics from University of Gießen, Germany in 1994. Since November 2012, she is a professor at RWTH Aachen University, Germany and since 2022 a guest professor at the department of engineering at Lund University, Sweden. Regina Dittmann is currently leading a group at Peter Grünberg Institute (PGI-7) in Forschungszentrum Jülich, working on atomically controlled growth of oxides, memristive devices, and neuromorphic circuits. She is an internationally recognized expert on memristive devices and the elucidation of their working and failure mechanisms.
Memristive devices are highly promising for neuromorphic computing owing to the significant latency and energy consumption improvement potential compared to existing hardware solutions. Their ability to be programmed to multiple resistance states can facilitate the realization of matrix-vector multiply operations within one step by exploiting Kirchhoff’s circuit laws. The accumulative property of the resistance of memristive devices can be utilized to implement artificial neuron network training and can be exploited for realizing local learning rules in spiking neural networks.
Certain memristive material stacks have been reported to exhibit analog switching at least in a limited dynamic range. In this talk, we discuss the relation between the switching dynamics of redox-based memristive devices and their analog programming capability. Theoretical considerations suggest that analog switching can be achieved if a thermal runaway is avoided. Moreover, we argue that internal series resistances play a crucial role in controlling the runaway and determining the accessible resistance window. Experimentally, we compare three groups of devices based on the valence change mechanism with characteristic properties, namely devices with small filaments (HfO2-TiOx) large filaments (SrTiO3) and area-dependent devices (Al/Al2O3/Pr07Ca03MnO3). We will explain the underlying switching mechanism and present the switching kinetics and discuss the possibility for linear and analog resistance programming.
Based on theoretical considerations and experimental analysis we identify general pathways for engineering gradual switching in valence change memories: (i) applying pulses shorter than the transition time, (ii) suppressing thermal runaway by systems showing larger filaments, (iii) including series resistances (ideally nonlinear) for suppressing the thermal runaway, (iv) using area-switching systems showing no Joule heating.
Westminster-I1
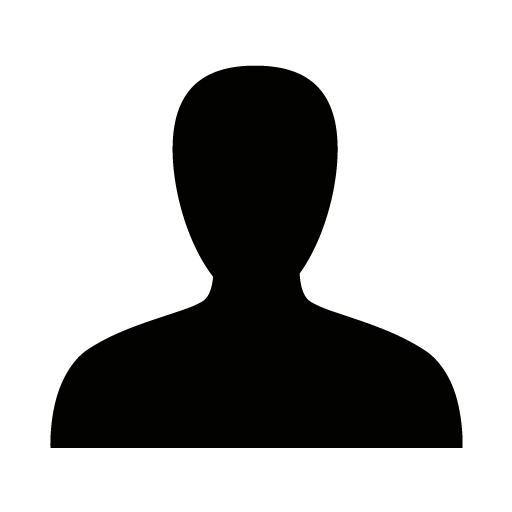
Redox-based resistive memory devices, also known as memristors or ReRAM, are highly promising elements for embedded nonvolatile memory, in-memory computing, and neuromorphic computing. Such devices switch resistance states through the electrochemical migration of oxygen vacancies in transition metal oxides. In this talk, we present our recent research on the materials thermodynamics of ionic motion in these oxygen-based memristors. Using a combination of device measurements, materials characterization, and multiscale physical modeling, we find that oxygen vacancies do not obey Fick’s First Law of diffusion as conventionally believed, but instead undergo composition phase separation This phase separation may result in chemical diffusion against the concentration gradients, thereby enabling certain filaments to be stable indefinitely from a thermodynamic perspective and would enable such devices to retain information for long periods. Finally, we utilize this understanding of phase separation in transition metal oxides to engineer retention time in a new class of three-terminal electrochemical memory. Our work shows the critical role of defect-chemical relationships in enabling ionic devices with the necessary performance metrics.
Westminster-O1
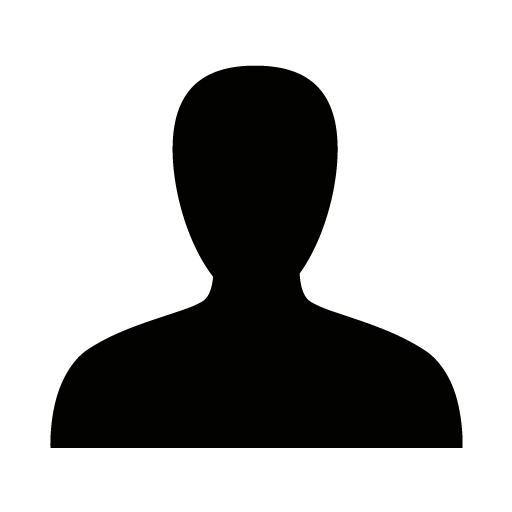
Mixed ionic and electronic conducting oxides, such as WO3, MoO3 or V2O5 are suitable candidates for programmable resistors in crossbar arrays, one of the most promising novel computing architectures for implementing artificial neural networks with high energy efficiency [1],[2]. By intercalation and distribution of mobile ions, such as H+, Li+, or Mg2+, the electronic conductivity of these materials can be finely tuned over a wide range, hence facilitating a high degree of control over the resistance state of each device [3],[4]. To reach target values for the operation speed of these so-called electrochemical ionic synapses, fast ion redistribution, and thus fast ion migration in these mixed conducting oxides is a key requirement [2].
In this contribution, we present our recent results from computational investigations of ion migration in these mixed conducting oxides with density functional theory (DFT) methods. We investigated the most favorable migration pathways with a particular focus on the effect of polarons on the proton mobility, which are the predominant type of mobile electronic charge carriers in these materials at low degrees of ion intercalation. Ultimately, aiming at accelerating ion migration and consequently the resistance modulation in electrochemical ionic synapses, we discuss methods to improve ion mobility in these mixed conducting oxides. Inspired by strategies to accelerate ion diffusion in high-temperature oxide ion conductors [5],[6],[7], we investigated lattice strain as a tool to tailor the migration characteristics for different ions and found significant potential to reduce migration barriers for intercalated ions in channel materials.
St.James-K1
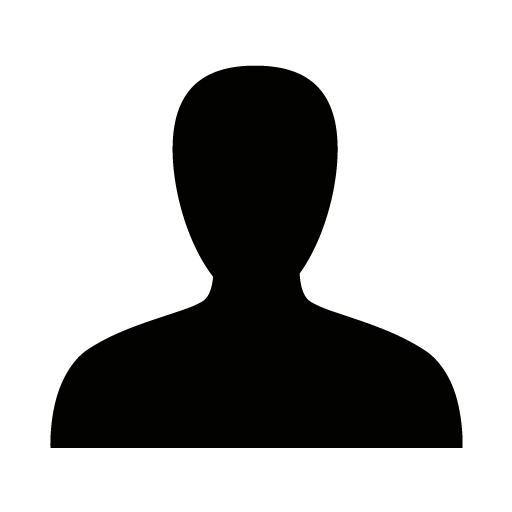
Protonic ceramic fuel and electrolysis cells (PCFC, PCEC) require fast kinetics of the oxygen reduction/water oxidation reaction at the air electrode (positrode). To extend the reactive zone beyond the three-phase boundary, positrode materials are desirable which combine mobile electronic defects, oxygen vacancies and protons ("triple conductors"). Because of the more covalent TM-O bonds, triple conducting (Ba,Sr)(Fe,Co,Zn,Y)O3-d perovskites typically show a lower degree of hydration than Ba(Zr,Ce)O3 electrolyte materials (see e.g. [1,2,3]).
Owing to the dominating electronic and competing oxygen vacancy conductivity, the experimental determination of the proton conductivity and mobility of (Ba,Sr)(Fe,Co,Zn,Y)O3-d positrode materials is challenging. The experimental approaches comprise water chemical diffusion measurements, and measurements of deuterium concentration profiles by secondary ion mass spectroscopy (SIMS). They show that proton mobilities in Ba(Fe,Y)O3-d are of comparable magnitude as in Ba(Zr,Ce)O3 electrolytes. However, the results also indicate that dopants such as Y3+ may lead to proton trapping effects in barium ferrates.
To obtain a detailed picture at atomistic level, proton migration in BaFeO3-d is also investigated by DFT calculations.[4] The proton migration barriers are found to depend both on the initial O...O and O-H distances. Interestingly, oxygen deficiency and correspondingly decreased formal Fe oxidation state lower the barriers. Proton barriers calculated with Sc3+ and Y3+ dopants further elucidate electrostatic and lattice distortion contributions to proton trapping. The characteristics of the proton transfer - being assisted by phonons providing a suitable O...O distance - is demonstrated by ab initio molecular dynamics.
Depending on the positrode morphology (dense thin film or porous thick film), the transport of oxygen vacancies may also become relevant for the overall surface reaction rate. Measurements in moderately reducing conditions (pO2 = 10-10 to 10-20 bar in an oxygen pumping cell) indicate that oxygen vacancies may also be affected by trapping at dopants in BaFeO3-d. Furthermore, the effect of dopant concentration on vacancy trapping may differ from the respective variation for protons (cf. related investigation for Ba(Zr,Y)O3-d [5]).
Overall, the detailed understanding of proton concentration and mobility as function of the specific materials composition is a key element for the rational optimization of PCFC/PCEC positrode materials, which have to fulfil mutually conflicting requirements (protonic and electronic conductivity, catalytic activity, long-term stability).
St.James-I1
Yue Qi is the Joan Wernig Sorensen Professor of Engineering at Brown University. She received her Ph.D. from Caltech in 2001. She spent the next 12 years working at General Motors and transitioned back to academia in 2013 as a faculty member at Michigan State University before joining Brown in 2020. She develops multi-scale simulation methods, starting from quantum mechanics, to design materials and interfaces for energy efficiency and sustainability. She has served in multiple inaugural roles aimed at increasing diversity in Engineering.
Entropic stabilized ABO3 perovskite oxides promise novel applications, including solid oxide fuel cells, catalysts, and two-step solar thermochemical hydrogen (STCH) production. While mixing multiple cations offers a new compositional space with vast tunability, it also introduces new computational challenges. These challenges include how to sample the structures within the size scale of Density functional theory (DFT) calculations; whether the structures are fully random or with local order; and how to define oxygen vacancy and its distributions in CCPO.
We calibrated our DFT-based predictive models with experiments in two CCPO systems, multiple A-site mixed {A}FeO3 and B-site mixed (La0.75Sr0.25){Mn0.25Fe0.25Co0.25Al0.25}O3 (MFCA). DFT was combined with the Metropolis Monte Carlo method (DFT-MC) to efficiently sample the possible cation configurations in CCPO materials. By comparing four different DFT-informed statistical models with experiments, we show that both the oxygen vacancy formation energy distribution (Evf) and the vacancy interactions must be considered to predict the oxygen non-stoichiometry (δ) accurately. Similar to experiments, the predicted δ can be used to extract the enthalpy and entropy of reduction through the Van't Hoff method, providing direct comparisons with experimental results. This procedure provides a full predictive workflow using DFT-MC to obtain possible local ordering or fully random structures, understand the redox activity of each element, and predict the thermodynamic properties of CCPOs, for computational screening and design of these CCPO materials at STCH conditions.
Our calculations also revealed several important characteristics of CCPO. First, as more cation types, especially above four, are mixed, the cell lattice becomes more cubic-like but the local cation-O octahedrons are more distorted. The oxygen vacancy did not show preferred site preference in the A-site {A}FeO3, but it is very sensitive to the B-sites. In the B-site mixed MFCA, the DFT-MC simulations predicted a significant increase in the local site preference of the cations (short-range ordering) in the presence of oxygen vacancies (VO), compared to a more random mixing without VO. Co is found to be the redox active element among other species (e.g. Mn, Fe), agreeing with experiments. In MFCA, Mn is the element that takes a 4+ oxidation state to compensate for the charge neutrality, while others are 3+. In the locally deformed lattice, the Fe-O are compassed, while Co-O bonds hold the most elongation, followed by Al, which provides extra mixing entropy that stabilizes the system. Thus, it was concluded that the VO prefers to be generated next to Co due to stretched Co-O bonds.
For ideal STCH applications with higher hydrogen production levels, the Evf distribution enabled positive Δδ (difference of δ between the two-step conditions) in the lower Evf and higher temperature range, where the traditional dilute and no-distribution model often misinterprets. This suggests the efficiency of water splitting and hydrogen production can be thermodynamically improved by mixing cations in CCPO and tunning both the average Evf and its distribution.
St.James-O1
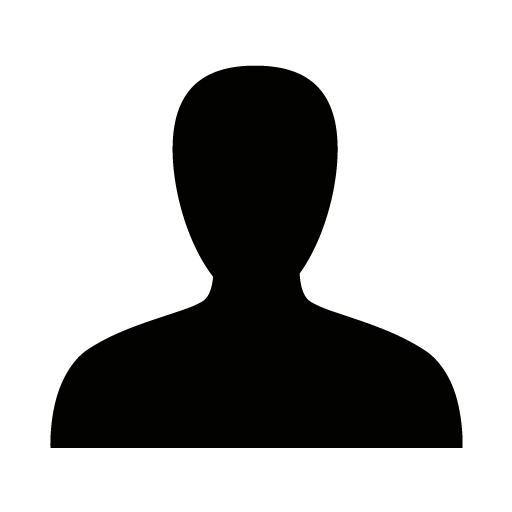
Triple-conducting oxides (TCOs) enable simultaneous transport of three charge carriers (h+/O2-/H+), and as a result are highly effective as positive electrode materials in a variety of electrochemical devices. Among prominent TCOs, the perovskite BaCo0.4Fe0.4Zr0.1Y0.1O3-δ (BCFZY4411) has garnered heightened interest due to its convenient synthesis and stability. Recent studies emphasize that the electronic/ionic transport behavior and electrocatalytic activity of this material can be further tailored through the Co/Fe composition. The Co and Fe doping not only modulates the ionic and electronic conduction behavior, but also enhances the catalytic activity for the oxygen reduction and oxygen evolution reactions (ORR/OER).
In a previous study, we investigated composition-dependent trends in the oxygen ion kinetics in the BCFZY system by adjusting the Co/Fe ratio [1]. We found that high Co content increases both the bulk oxygen ion diffusivity and the oxygen surface exchange kinetics. However, the effect of the Co/Fe composition on bulk proton diffusion and surface exchange remains unresolved. Although the proton kinetic behavior in BCFZY4411 has been partially examined, studies have been limited to electrical conductivity relaxation (ECR) investigations [2-4], which cannot unambiguously isolate the proton dynamics due to the presence of multiple charge carriers. Therefore, limiting assumptions and complex analyses are generally required to decouple the dynamics of oxygen ions and protons when using the ECR technique.
Here, we combine a series of alternative characterization techniques, including isotope-labeled time-of-flight secondary ion mass spectrometry (TOF-SIMS), isotope-contrast powder neutron diffraction (ND), and solid-state nuclear magnetic resonance (SSNMR) to untangle the proton local environments and dynamics in the BCFZY system. First, the isotope exchange technique was used to determine tracer proton bulk and surface kinetic properties by selectively exchanging isotopic species (H2O/D2O) in dense BCFZY pellet samples across a range of temperatures. ToF-SIMS was used to collect data on OH-/OD- species, and the isotope diffusion profiles were fitted by the diffusion solution to obtain proton tracer diffusivities and surface exchange coefficients as a function of BCFZY composition and temperature. Secondly, temperature-resolved ND experiments coupled with pair distribution function (PDF) analysis was used to analyze the crystallographic features of dried, hydrated, and deuterated BCFZY samples. Proton locations and concentrations in the BCFZY structure were established using Fourier difference maps, and activation energies for proton migration in the local structure were estimated based on bond valence sum analysis. Finally, SSNMR was employed to quantify the proton content in the bulk and surface regions of hydrated BCFZY samples. While the bulk proton concentration decreased with increasing Co content, the surface proton content showed the opposite trend. Wide-line analysis was utilized to study proton mobility, and a higher Co-content exhibited lower proton mobility. This study provides a comprehensive insight into proton kinetics in the BCFZY system through combined characterization approaches and can guide the understanding and design of other Co and Fe-containing TCOs.
Moore-K1
Prof. Jennifer Rupp is the Thomas Lord Associate Professor of Electrochemical Materials at the Department of Materials Science and Engineering, and Associate Professor at the Department of Electrical Engineering and Computer Science at MIT. Prior she was a non-tenure track assistant professor at ETH Zurich Switzerland where she held two prestigious externally funded career grants, namely an ERC Starting Grant (SNSF) and Swiss National Science Foundation (SNF) professorship.
She previously was affiliated as a visiting and senior scientist at MIT (2012-2011), the National Institute of Materials Science (NIMS) in Tsukuba, Japan (2011), and was working as a postdoc at ETH Zurich (2010-2006). Rupp team`s current research interests are on processing of ceramic and glass materials, solid state material design and tuning of structure-property relations for novel energy and information devices and operation schemes. This ranges from alternative energy storage via solid state batteries, solar-to-synthetic fuel conversion or novel types of neuromorphic memories and computing logic entities for data storage and transfer beyond transistors and new sensing functions to track chemicals in the environment. Here, her team goes the whole way from material design, novel processing techniques to make ceramics, cermets or glassy-type ceramic structures up to novel device prototypes, their operation and characteristics.
She has published 90 papers, holds 12 patents, and, being a frequent speaker and panel member of the World Economic Forum, enjoys discussing material tech trends on the theme of energy with the public, economists and policy makers. Rupp also enjoys engaging with companies all around the world through both consultancy and collaborations focused either on material processing business or electrochemical device & product engineering (e.g. battery, oil & fuel processing, sensing, electronic companies). Currently, she holds a position as associate editor at Journal of Materials Chemistry A and is also on the advisory board member for Advanced Functional Materials and Advanced Materials Interfaces.
Rupp and team received several honors and awards such as the Displaying Future Award by the company Merck KGaA 2018 for a glucose converting fuel cell chip, BASF and Volkswagen Science Award 2017 for battery research, "Top 40 international scientist under the age of 40" by World Economic Forum 2015, Spark Award for the most innovative and economically important invention of the year 2014 at ETH Zurich, Kepler award “new materials in energy technology” by the European Academy of Science 2012 or Young Scientist Award by the Solid State Ionic Society. She gave keynote lectures at Royal Society UK 2018, Nature Energy conference 2016, Gordon Research lecture 2014 and many others, also she presented on battery and energy technologies at the World Economic Forum 2017.
Lithium, Interfaces & Action:
Designing Next Solid Battery Materials –
From Engineered Grain Boundaries to Novel High Entropy Amorphous Phases
Jennifer L.M. Rupp
Technical University of Munich & TUM International Energy, Germany
jrupp@tum.de
Next generation of energy storage devices may largely benefit from fast and solid Li+ ceramic electrolyte conductors to allow for safe and efficient batteries. For those applications, the ability of Li-oxides to engineer their interfaces and be processed as thin film structures and with high control over Lithiation and phases at low temperature is of essence to control conductivity. Through this presentation we review the field from a new angle, not only focused on the classics such as Li-ionic transport and electrochemical stability window for Li-solid state battery electrolytes, but focusing on opportunities and challenges routes in thermal and ceramic processing of the components and their assemblies with electrodes. Also, we will carefully review and give perspectives on the role of solid state battery ceramic strategies for the electrolyte on the electrode interfaces and towards charge transfer and vs. current densities.
In the first part we will look at various options to either eingineer grain boundaries as a way to control majority and minority charge carriers at interfaces and within space chages to ultimately alter critical current densities of batteries. Or, in the opposite second part synthesize and design a new class of ‘high entropy” Li amorphous conductors without any grain boundaries. Both material cases will be demonstrated based on Li-garnets that have so far the highest known number of local bonding units and a rich nature to either manipulate the amorphous Li+ conductance or grain boundaries via dopants. Collectively, the insights on solid state energy storage provide evidence for the functionalities that those Li-solid state material designs can have in new materials and synthesis for cost and mass manufacturable solid state and hybrid battery prototypes.
Moore-I1
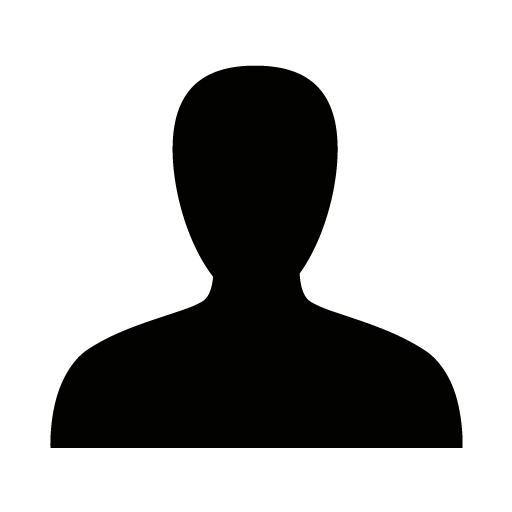
Meeting the escalating energy demands of electric vehicles and portable devices necessitates high-energy rechargeable batteries. Although Li-ion batteries are prevalent, they face challenges related to energy density, charging rates, and safety concerns associated with flammable liquid electrolytes. To address these issues, researchers are exploring alternative batteries or investigating solid-state electrolytes as a safer alternative to conventional Li-ion batteries. Among various inorganic materials garnering attention in solid-state battery systems, Li-La-Ti-O (LLTO) stands out as a promising candidate as it exhibits high bulk Li ion conductivity, excellent high voltage stability, and remarkable hardness, which mitigates dendrite formation. Consequently, there have been several efforts to improve their conductivity. Literature suggests that the low conductivity of LLTO is due to grain boundaries, while conversely, LLTO conductivity increases in the presence of TiO2. Both effects, the negative impact of grain boundaries and the positive influence of TiO2 lack a satisfactory explanation, prompting us to perform a comprehensive Molecular Dynamics investigation. Our study involves a detailed analysis of symmetric and mixed LLTO grain boundaries and LLTO/TiO₂ interfaces under diverse conditions. This exploration has yielded intriguing insights into the complex interplay of these factors. In this presentation, I will share our results and delve into the intricate migration mechanisms of Li-ion within the elaborate microstructures of LLTO. These findings not only shed light on the mysterious phenomena of LLTO conductivity but also offer valuable perspectives for designing and optimizing solid-state batteries, shaping the future of energy storage applications.
Moore-O1
The excess resistivity of grain boundaries in ion-conducting oxides is generally attributed to the presence of space-charge layers in which oxygen vacancies are depleted. Acceptor cations are commonly assumed to take a uniform concentration profile throughout the sample (Mott–Schottky case). This simplification enables a simple analysis of impedance data with closed-form expressions, but it disregards that acceptor cations will typically be able to accumulate within the space-charge layers at sintering temperatures, at which the acceptor cations are mobile. Upon cooling, the immobilised acceptors will then retain an accumulated profile. This aspect is taken account of by the restricted-equilibrium model.
In this work, we explore the effects of restricted equilibria on grain-boundary properties by means of continuum simulations. Our implementation of a drift–diffusion framework allows for the calculation of impedance spectra for space-charge scenarios with a realistic thermal history, using acceptor-doped CeO2 as a model system. These results reveal the errors that arise from analysing complex space-charge situations with the simplified Mott–Schottky expressions. We also discuss how fundamental differences between elevated-temperature and room-temperature experimental techniques may arise, in the model electroceramic SrTiO3, from the influence of restricted equilibria.
Gielgud-K1
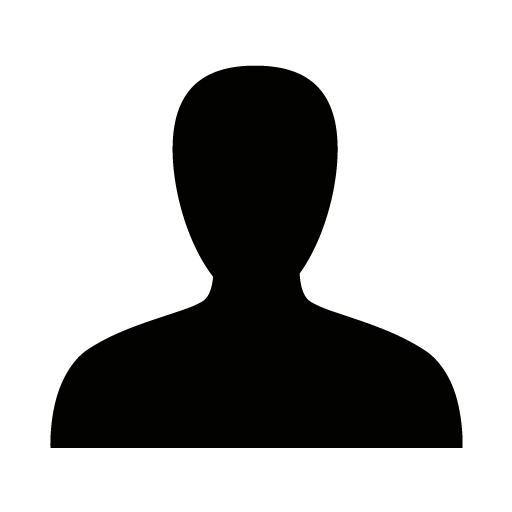
Solid oxide fuel cell (SOFC) is all-solid-state energy conversion device that converts directly chemical energy stored in fuel into electricity. Significant efforts have been devoted to developing high-performance electrode materials with the aim of increasing the power density of SOFC stacks. Besides electrochemical activity, the structural stability of electrode material is equally important for practical long-term operation of SOFCs. Compared to single perovskite, B-site perovskites have low thermal expansion coefficient and high structure stability. However, they usually show unsatisfied electrochemical activity as SOFC electrode materials. In this work, we modulate the electrode reaction kinetics by constructing anti-site defects in the B-site double perovskite lattice through the design of element occupation, which promote the formation of electronic and oxide ionic conduction network, and so facilitate the charge transport through the electrode. On the other hand, we constructed heterogenous coherent interface in B-site and simple perovskite composite cathode to accelerate the charge transport through the formation of interface tensile stress. The inherent interface limits the chemical expansion and element segregation, enhancing the structure stability. These two works demonstrate the great potentials of B-site perovskite in high performance electrodes for SOFCs.
Gielgud-I1
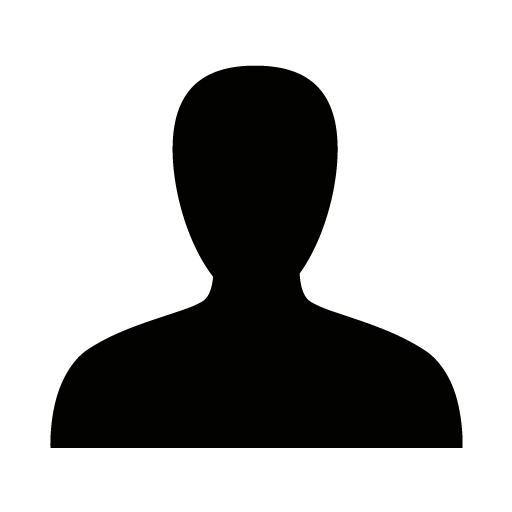
The biggest drawback of graphene is its resistance for ion adsorption, which results in a limited ability to store ions. There are methods described in the literature that can alter graphene's structure and impact its inert behavior. Typical of these methods are creating point and line defects, adding extra atoms, and substituting carbon atom with another one.
Recently, there has been studies to replace the carbon atoms of graphene with germanium. The so called germagraphene is a two-dimensional material composed of germanium and carbon atoms arranged in a honeycomb lattice. Germagraphene has a higher theoretical capacity than the graphene due to the presence of germanium atoms, which can increase the number of lithium ions that can be stored in the material. It also has higher electronic conductivity than graphene due to the presence of germanium atoms, which can facilitate the transport of electrons during battery charging and discharging processes. The germanium atom creates an active site in the structure and therefore contributes to the improvement of battery performance by enhancing the electron transfer at the electrode-electrolyte interface. Overall, germagraphene's unique properties make it a promising material for battery applications, particularly as a cathode material in dual-carbon batteries (DCBs), which are low-cost and environmentally friendly alternatives to conventional lithium-ion batteries.
The reversible coupling of ions at two carbon-based electrodes forms the foundation of the energy storage technology known as DCBs. The lack of appropriate cathode materials, however, restricts the development of high-performance dual-carbon batteries. An intriguing cathode material for dual-carbon batteries has been reported by our group in a recent publication entitled “First-Principles Investigation of Charged Germagraphene as a Cathode Material for Dual-Carbon Batteries” [1]. Charged germagraphene was investigated as a potential cathode material using first-principles techniques to examine its electrochemical characteristics. First-principles calculations using density functional theory (DFT) were employed to evaluate the electronic properties of graphene and germagraphene for both under neutral and electrically biased conditions. The effective screening method (ESM) method was used to calculate these properties under electrical bias [2].
In this presentation, the atomistic picture of germagraphene as a cathode material that outperforms graphene in terms of electrochemical performance will be drawn. The enhanced anion adsorption on germagraphene is due to its reduced work function, higher charge carrier mobility, and redox potential. The research also showed that anions are easily adsorbed onto the surface of the charged germagraphene. The results demonstrate a significant potential of charged germagraphene as a positive electrode material for sustainable energy storage systems.
Gielgud-O1
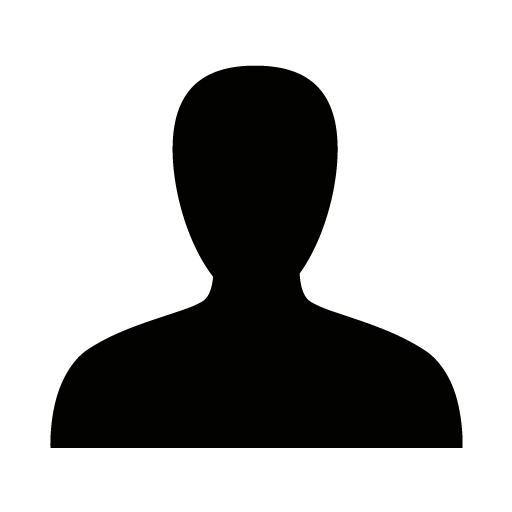
Solid-state ion conductors (SSICs) play an important role in electrochemical energy storage devices, such as all-solid-state batteries,[1] due to improved safety and high energy density. Considering the plethora of available SSIC candidate materials,[2] high-throughput experiments and simulations are essential in the search for suitable SSICs that offer high ionic conductivities. First-principles methods are the conventional approach to investigate the interplay between the anharmonic host lattice and mobile ions on an atomic level,[3,4] while being limited to few selected systems due to the large computational costs.
Here, we systematically explore the potential of machine-learning molecular dynamics (MLMD) for the prediction of the complex ion migration mechanisms including anharmonic vibrational properties in the host lattices.[5] We choose three classes of SSICs in which different mechanisms govern the migration of ions, and which all include vibrational anharmonicities: AgI, a strongly disordered Ag+ conductor; Li10GeP2S12, showing concerted Li+ migration; and tungsten-doped Na3SbS4, a Na+ vacancy conductor. These materials represent a variety of challenges for MLFFs including anharmonicities, dynamical potential energy surfaces as well as rare hopping events. Systematic comparison with ab initio molecular dynamics simulations reveals that MLMD captures accurately anharmonic vibrational properties in the host lattice and particularly is able to predict the underlying physical mechanism that governs ion migration. We will finally discuss the potential of MLMD simulations to complement experimental techniques in the investigation of finite-temperature vibrational properties and, thereby, to enable the rapid design of novel SSICs.
Fleming-I1
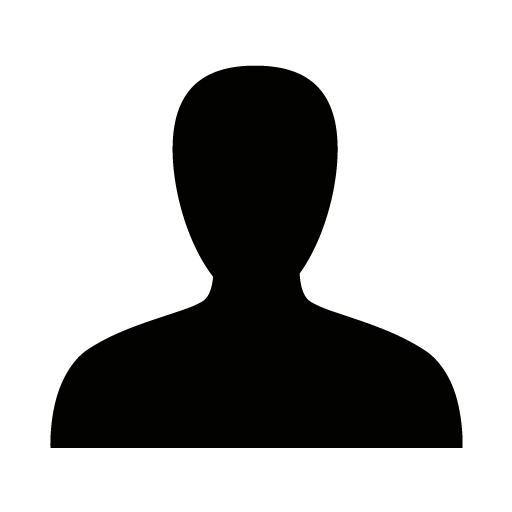
In recent decades, tuning the anionic sublattice in oxides with hydrogen, nitrogen, fluorine, chlorine etc., is attracting growing interests. Among them, fluorine with the similar ionic radius as oxygen ions and able to occupy the oxygen sites in the lattice bringing anionic disorder, is of particular interest for applications in solid oxide cells (SOCs) or oxygen transport membranes (OTMs). Moreover, its lower electronic charge is expected to result in the concomitant reduction of the oxidation state of transition metal cations to maintain the electronic neutrality bringing changes in electronic behaviour. The strong electronegativity of F is predicted to destabilise the M-O bond, potentially beneficial for increasing oxygen diffusion kinetics. Previous investigations [1,2] on different oxide materials have demonstrated that a small amount of F doping is capable of improving the electrochemical and oxygen transport performance for materials applied in high temperature devices. Despite of the promising results, thermal stability of the F-doped materials is scarcely investigated considering the material preparation and device operation are both at elevated temperatures. Furthermore, post mortem chemical analysis on the materials to verify whether F stays in the oxide lattice after the high temperature annealing and studies on the mechanism of the improved electrochemical performance, are even rarely seen.
In this work, a state-of-art mixed electronic and ionic conducting (MIEC) material La0.6Sr0.4Co0.2Fe0.8O3-δ (LSCF6428) as the oxygen electrode in the SOCs was chosen as the parent oxide material. The F-doped La0.6Sr0.4Co0.2Fe0.8O3-δFx powders (LSCFFx, x = 0.05, 0.10 and 0.20) was obtained via low temperature fluorination with PVDF binder. The incorporation of F in the oxide lattice has been carefully studied via X-ray diffraction (XRD), X-ray Photoelectron spectroscopy (XPS) measurements. The incorporated F concentration was further quantified with the fluoride selective electrode. Symmetric cells were prepared with LSCF and LSCFFx materials, and the cell performance was characterised with electrochemical impedance spectroscopy. Furthermore, the thermal stability of the LSCFFx oxyfluoride has been carefully studied with TGA-MS measurement and a comprehensive post mortem chemical analysis has also been carried out with various techniques. The absence of F is confirmed for the symmetric cells while an improvement in electrochemical performance is indeed observed. The oxygen surface exchange behaviour of the LSCFF materials with verified fluorine presence was further studied via pulsed isotopic exchange (PIE). The results raise the doubt if the fluorine incorporation is truly beneficial for the electrochemical performance of the materials with applications in solid oxide cells. Structural and chemical investigation (XRD, Mossbauer spectroscopy, and XPS analysis) were carried out on the materials after fluorine loss to understand the effect of preliminary PVDF treatment on the electrochemical properties. The main results of this work will be detailed during this presentation.
Fleming-O1
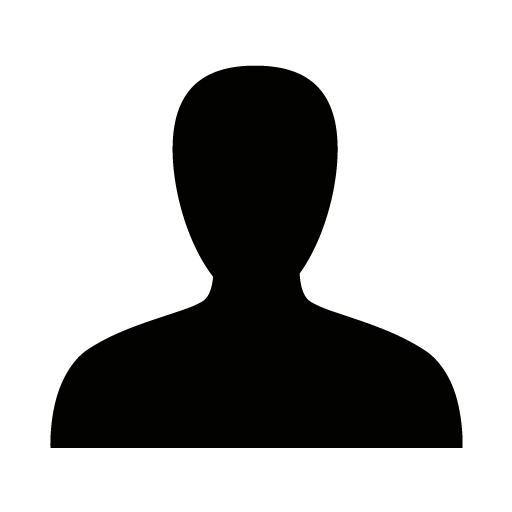
Introduction
For highly efficient hydrogen generation by water electrolysis, enormous efforts have been devoted to the development of oxygen evolution reaction (OER) catalysts. Although cation doping is a general design strategy, its effectiveness is reaching the limitations and alternative strategies are demanded. Anion defect engineering is one of promising strategies for the development of oxide-based OER catalysts. For instance, OER activity of BaFe1-xCoxO3-δ were improved by substituting part of O with F.[1] To realize flexible control of anion composition, we developed a new anion-doping technique by using an electrochemical reactor and succeeded electrochemical F-doping into a perovskite oxide La0.5Sr0.5CoO3-δ (LSC55).[2] In this study, by evaluating the OER activity of electrochemically F-doped LSC55, we aimed to elucidate the influence of F defect on OER activity.
Experiment
LSC55 was synthesized by Pechini method. For the F-doping to LSC55, the electrochemical cell, LSC55-BaF2|BaF2|PbF2-Pb, was fabricated. Here, BaF2 and PbF2-Pb were a fluoride-ion conductor and an F source respectively. 20 mol% of F was electrochemically supplied into LSC55 under the constant current 60 μA/g at 250°C. The crystal structure and surface morphology of the prepared samples were characterized by x-ray diffractometry (XRD) and annular bright field scanning transmission electron microscopy (ABF-STEM). To check the existence of F in the sample, x-ray photoelectron spectroscopy (XPS) F1s spectrum was measured.
For the OER test, the catalyst ink was prepared by mixing the catalyst powder, K+-exchanged Nafion and tetrahydrofuran. 6.4 μl of the catalyst ink was sonicated and dropped onto the glassy-carbon rotating disk electrode. Electrochemical measurement was performed with a Hg/HgO reference electrode and a Pt wire counter electrode in O2-saturated 0.1 M KOH. To evaluate the OER activity, cyclic voltammetry (CV) was performed from 0.3 to 0.9 V at 10 mVs-1 with a rotation rate of 1,600 rpm. The current density was normalized by the surface area of the disk electrode.
Results and discussion
By XRD measurements, it was revealed that the crystal structure of both the pristine and the F-doped LSC55 was a rhombohedral perovskite structure (space group: R3c ). An XPS F1s peak was clearly observed from the F-doped LSC55, which supports successful F-doping on the surface of the sample. The ABF-STEM observation on the pristine sample revealed both the surface and the bulk region maintain highly crystalline state. On the other hand, surface layer composed of amorphous and nano-size crystalline domains was observed in F-doped LSC55 particles.
OER activities were evaluated by CV. The initial OER activity of the F-doped LSC55 was higher than that of the pristine. The reaction current at 1.8 V of the F-doped LSC55 was about 12% higher than that of the pristine. Moreover, the F-doped LSC55 showed better stability upon CV cycles than the pristine. The degradation rate upon 100 CV cycles of the F-doped LSC55 was 83% while that of the pristine was 61%. These indicate that electrochemical F-doping can improve both catalytic activity and its stability.
In the presentation, the influence of the F-doping condition (F concentration, F-doping rate) on the OER activity of LSC55 is discussed.
Fleming-O2
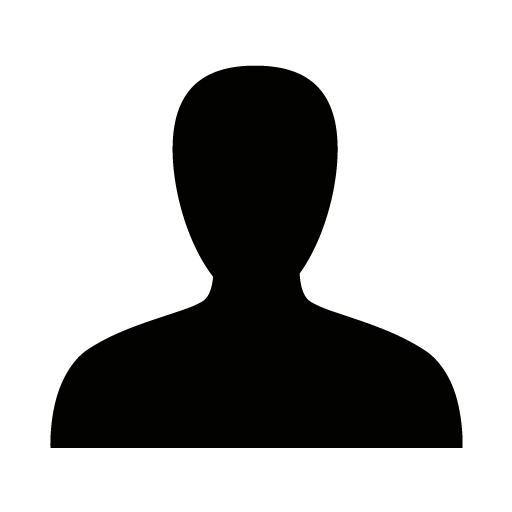
Solid oxide cells (SOCs) show great potential for green hydrogen production and energy conversion, yet their limited durability remains a bottleneck to their widespread commercialisation. This study investigates the impact of humidity on oxygen electrode degradation, using a state-of-the-art cell architecture of LSCF|LSCF-GDC|GDC|YSZ|Ni-YSZ. Long-term durability tests were conducted at 800°C under varying humidity content (dry, 3%, and 8%) in both electrolysis (SOEC) and fuel cell (SOFC) operating modes. Electrochemical impedance spectroscopy (EIS) measurements were performed to assess cell performance and identify degradation mechanisms before and after extended testing.
EIS analyses revealed a notable increase in the medium-frequency semicircle of the oxygen electrode during humid SOEC operation compared to dry condition, indicating electrode degradation (Figure 1-a). All the tested cells showed an increase in high frequency arc, which was attributed to hydrogen electrode degradation [1]. The increase in ohmic resistance classically observed under dry SOEC mode was found to be aggravated under humid condition. Post-test characterizations identified a clear elemental inter-diffusion across various cell layers, which was observed in all samples already after cell manufacturing [1]. The degradation of the hydrogen electrode was mainly caused by Ni depletion and coarsening in the SOEC mode under dry air conditions. The degradation of the oxygen electrode was only noticeable during ageing in humid air conditions, resulting in the formation of a dense Sr oxide layer on the top surface of the current collector layer (Figure 1-b) [2]. To complement the experimental findings, The Density Functional Theory (DFT) method was employed to calculate the energy of LSCF decomposition according to different scenarios under dry and humid conditions. The results provided insights into the Sr oxide growth in humid vs dry air conditions.
The study highlights the detrimental effect of humidity on oxygen electrode degradation in SOCs, particularly in SOEC mode. The identification of Sr oxide formation as a key factor in oxygen electrode degradation under humid conditions paves the way for targeted strategies to mitigate this issue and enhance SOC durability for practical applications.
[1] G. Sassone et al., Effect of the Operating Temperature on the Degradation of Solid Oxide Electrolysis Cells. submitted.
[2] G. Sassone et al., Impact of Air Moisture and Operating Mode on the Degradation of Solid Oxide Cells. In preparation.
Fleming-O3
Tae Ho Shin (Tim. J. Shin) is a Director for Low Carbon & DX R&D division and Head of Hydrogen Energy Materials Centre at Korea Institute of Ceramic Engineering & Technology (KICET), South Korea. He was born in Daejeon, South Korea. He received M.S. in ceramic engineering from Yonsei University and his PhD in advanced materials chemistry from Prof. Tatsumi Ishihara at Kyushu University (PhD 2012), Japan. He held a research fellow position, followed by University of St Andrews Research Fellowship (2012-2015) and worked with Prof. John T. S. Irvine. After fellowship work with Prof. John Irvine at School of Chemistry in University of St Andrews, he joined the researcher of Energy Materials Centre at KICET, Korea. His research involves developing new oxide electrode for the electrochemical devices such as SOFC and SOEC. An area of particular interest is crystal structure analysis for understanding and predicting the design of materials with targeted electrochemical properties. As a member of the committee for RPS-REC in KOREA, KETEP’s Fuel Cell R&D annual plan, and Korean Carbon Neutrality R&D roadmap(2021), he has been serving the related government ministry. Recently, he got the distinguished star researcher award from KICET (2021), outstanding technology KICET award (2017), and several domestic academic awards. Previously, he had received the Reaxys PhD Prize Finalist Award (2012), Dr. Bernard S. Baker Student Awards for Fuel Cell Research (2011, FCS&E USA), and the Electrochemical Society (USA)’s Summer Fellowship Award (2011).
Utilizing rare-earth doped ceria in solid oxide cells (SOCs) engineering is indeed a strategy to enhance the electrochemical devices' durability and activity. Gd-doped ceria (GDC) is actively used for barrier layer and catalytic additives in solid oxide fuel cells (SOFCs). In this study, we conducted experiments with La-doped CeO2 (LDC) or Bi-doped Ceria-based materials, in which the Ce sites are predominantly occupied by La, to prevent the formation of the Ce-Zr solid solution. This LDC was comparably used as a functional interlayer between the electrolyte and cathode if sintered at lower temperatures to avoid La2Zr2O7 impurity. In addition, the high substitution of La3+ into the ceria lattice improves the oxygen non-stoichiometry of LDC, leading to accelerated electrochemical high performance by the additional role of LDC for oxygen supplier capacitance at high-current operation. Thus, we confirmed that the improved SOFC high performance was achieved at the maximum power density (MPD) of ~2.15 W cm-2 at 800oC when the optimized LDC buffer layer was hired at the anode supported typed- Samsung’s SOFC by lowering the sintering temperature to prevent LDC’s impurity reaction.
References
[1], C. Graves, Nat. Mater., 2014, 14, 239-244
[2], Tatsumi Ishihara, J. Korean Ceram. Soc. > Volume 53(5); 201
Westminster-I1
Complex oxides have evolved as a major class of functional applied in a wide range of energy conversion and storage approaches as well as electronic devices, which harvest the ability to precisely tailor and combine oxides on the nanoscale. Here, miniaturization and confinement phenomena can occur that determine the ionic-electronic structure of oxide thin films, surfaces, and interfaces. Here, we will discuss how dedicated design and understanding of interfacial space charge phenomena can be used to tailor electronic and ionic charge transport. We will specifically discuss how solid state ionics phenomena in oxide thin films can be exploited to trigger structural, electronic, and magnetic phase transitions [1] and discuss means to control the dynamics of oxygen exchange in such nanoscaled oxides. Finally, we link space-charge phenomena along and across electrochemically active oxide interfaces, with particular focus on the role of space charge at solid-liquid interfaces operating in alkaline water splitting. We will discuss materials engineering strategies that allow to overcome the limitations of ‘bulk catalyst’ owing to intrinsic scaling relations and the typically observed inverse relationship of catalyst activity and long term stability. [2,3]
[1] S. He et al., Adv. Func. Mater., in press (2024)
[2] Weber et al., JACS (2022);
[3] Heymann et al., ACS Appl. Mater. Interf. (2022)
Westminster-O1
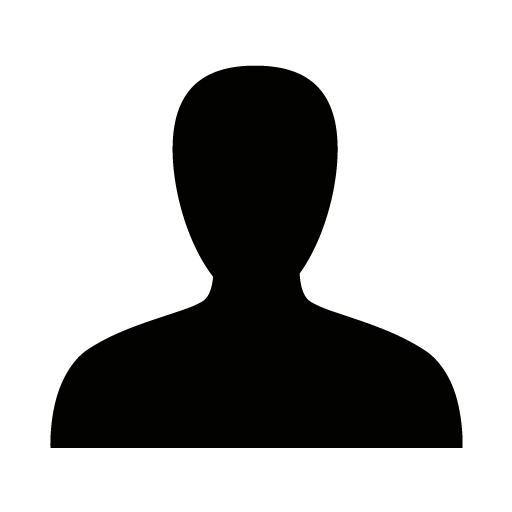
Enhancing the switching speed of oxide-based memristive devices at a low voltage level is crucial for their use as non-volatile memory and their integration into emerging computing paradigms such as neuromorphic computing. Memristive devices based on the valence change mechanism (VCM) have exhibited substantial potential not only in the domain of digital emerging memories but also in analog neuromorphic and in-memory computing applications. Specifically, filamentary ReRAM devices have been reported to exhibit significant temperature accelerated switching kinetics. Here we demonstrate a significant improvement in the speed of the SET process by engineering this thermal acceleration for the memristive model system SrTiO3.
We have implemented a novel approach of thermal engineering in the ReRAM device stacks, without altering the properties of the switching oxide or its interfaces. In this work we present two different variations: i) we have used reduced tantalum oxide as the main body of the active electrode, which is electrically conductive, but still has relatively low thermal conductivity (TaOx, κ ~ 5.9 W/m K), and ii) we have introduced an electrically insulating, low thermal conductivity interlayer (HfO2, κ ~ 0.6 W/m K), embedded inside the Pt (κ ~ 44.5 W/m K) top electrode of the device. In both approaches, the introduced materials act as a thermal barrier in the z-direction, which reduces the thermal conductance through the active top electrode.
By incorporating a heat blocking layer (HfO2 or TaOx) positioned within the active electrode of our STO model system VCM devices, we have been able to achieve a remarkable acceleration in switching speed, reaching up to 103 times faster in terms of time. In addition, this approach enables an approximate 30% reduction in the switching voltage required to sustain the switching speed. This not only improves the energy efficiency of ReRAM cells, but also positions them well for integration with low-voltage transistor technology. The choice of HfO2 or TaOx as heat barrier materials facilitates the implementation of our approach due to the wide availability of the materials and the simplicity of their fabrication process. The fact that there are no modifications of the main stack of the ReRAM device (ohmic contact/switching oxide/Schottky contact) makes this approach a more general mechanism to accelerate the switching kinetics in ReRAM devices and, not limited to the specific memristive SrTiO3 stack used in this work.
In conclusion, we were able to accelerate up to x103 time the SET speed process of filamentary ReRAM devices or reduce the operation voltage by up to ≈ 30% to maintain the switching speed, by thermally engineering the heat dissipation along the active metal electrode of the devices. This opens a window towards more efficient device operation with a strategy that is CMOS compatible, making our approach easily implemented in established processes of ReRAM device fabrication.
Westminster-O2
Artificial neural network (ANN)-based computing [e.g., deep learning with a multi-layer neural network (NN)] can provide excellent learning, classification, and inference characteristics that are close to, and in some cases beyond, those found in natural intelligence (i.e., the human brain), whereas the enormous amounts of power required by ANN (as in a typical multi-layer NN) are far higher than that required by human beings. To overcome the low energy efficiency of ANN computing, in-materio computing, in which inherent properties of materials are harnessed to perform computing, has recently been attracting attention. Among various types of the computing, physical reservoir computing (PRC) is particularly attractive because of its ability to significantly reduce the computational resources required to process time-series data by utilization of the nonlinear responses of a ‘reservoir’ (material or device, as a dynamical system) to input signals. Chaotic dynamics (including edge of chaos dynamics) has been theoretically predicted to be advantageous for achieving high performance PRC, because of excellent nonlinearity and high dimensionality (diversity) of output, which enable mapping input to high dimensional feature space. Realizing chaotic dynamics with nanomaterials and/or nanospace is thus a great challenge for development of low power consumption and highly integrated ANN-based computing devices. Recently, we have developed high performance PRC devices on the basis of nanoionics principle [1-4]. One example is a novel PRC nanoionic device, ion-gating reservoir, which utilizes ion-electron coupled dynamics in the vicinity of solid electric double layer in the edge of chaos state [1,2]. Various time-series data processing including nonlinear autoregressive moving-average (NARMA) task, hand written digit recognition, and spoken digit recognition could be performed with high accuracy. Redox behaviors of ion-electron mixed conductors (e.g., Li-WOx, LiCoO2) have also been successfully applied to ion-gating reservoirs, leading to slow responses, which are advantageous for dealing events with time constants over several tens of seconds [3,4]. In the presentation, the performance of the devices in various computing tasks, and the mechanisms will be discussed.
Westminster-O3
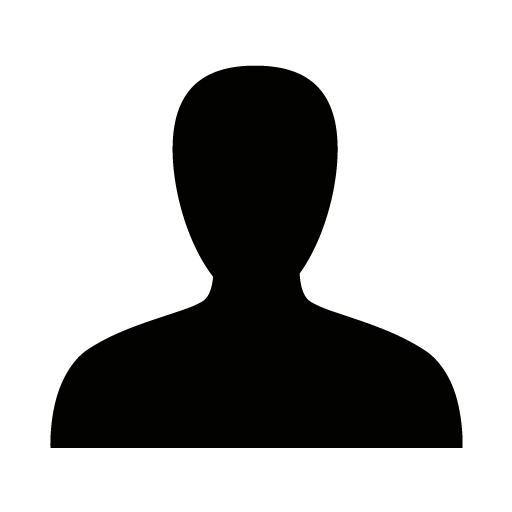
Philipp received his bachelor’s and master’s degree at University of Ulm (Germany) in the course Chemistry & Management. During his studies he worked on PEM fuel cells at Daimler Truck Fuel Cells Company (Master) and the Center for Solar Energy- and Hydrogen Research in Ulm (ZSW, Bachelor). After an internship in a consulting company (Camelot Mgmt. Consulting) he received the ERASMUS-scholarship and studied six months in Turku (Finland). Currently, he is a PhD student at the Catalonia Institute for Energy Research (IREC, Barcelona) focussing on electrolyte gated synaptic transistors.
The field of synaptic transistor research has been gaining attention for analog computing solutions in recent years. This is due to their potential of forming networks to overcome the von Neumann bottleneck, which is the fundamental limitation in traditional digital computing systems caused by the separation of data memory and processing units. Synaptic transistors rely on the ability to form junctions with each other that can be strengthened or weakened by external stimuli causing transport processes such as intercalation of ions. Mimicking the behavior of biological synapses in this way allows for advancements in energy efficiency and processor performance as well as new forms of computing, such as neuromorphic computing and artificial intelligence, inspired by the structure and function of the brain.
Among the different solutions suggested in literature, Electrolyte-Gated Transistors (EGTs) – which are essentially conventional Field-Effect Transistors (FETs) where the gate dielectric is replaced by an electrolyte with mobile ions – have recently been proposed. Many of these EGTs are based on the principle of ionic intercalation, utilizing the active kinetics of ions such as H+ or Li+. On the other hand, perovskite oxide materials hold large potential as EGTs due to their ability to be easily modified by oxide-ion intercalation, with oxide-ion insertion being more widely applicable than other types of ions. However, the drawback of the several orders of magnitude lower oxide-ion transport present a large drawback, especially in all-solid-state EGTs. The specific EGT studied in this research is a symmetric battery-like device in which oxide-ions are transferred between two mixed ionic-electronic conducting (MIEC) perovskite oxide thin-films (i.e. channel and gate) through a solid state electrolyte. In order to address the sluggish oxide-ion diffusivity, a superior oxide-ion conducting thin film of Bi2V1‑xCuxO(11/2)‑x (BICUVOX) with highest oxide-ion conductivities at low temperatures ever reported is used as low temperature electrolyte.[1] This EGT is manufactured by pulsed laser deposition (PLD), Photolithography and reactive ion milling. The conductance of the channel is controlled via a gate bias, allowing for oxide-ion intercalation between gate and channel as well as multistate operation in the millisecond range. In-situ electrical conductivity measurements at low temperatures (<150 °C) reveal fast and remarkable low energy consumption. Due to its multi-states, the presented EGT reveals synaptic features such as short- and long-term plasticity (STP, LTP), low asymmetric ratio during weight update and broad dynamic range which are known as fundamental properties of synaptic plasticity in biological neural networks. By this, the proposed synaptic transistor has the potential to lead to a breakthrough in energy efficiency and processor performance in information technology.
St.James-I1
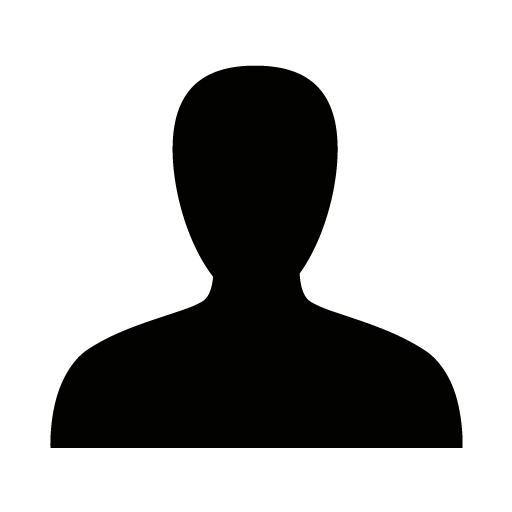
Oxygen vacancies play crucial roles in a wide range of interesting and important phenomena such as superconductivity, magnetoresistance, resistance switching, piezoelectricity, and oxide ion conductivity.
Presence of oxygen vacancies and oxide ion conductivity may play positive or negative roles for practical applications. For example, for electrochemical applications such as solid oxide fuel/ electrolysis cells and oxygen separation membranes, creations of oxygen vacancies and high oxide ion conductivity are essential to enhance device performances. For dielectric applications such as multilayer ceramic capacitors, however, oxygen vacancies can become mobile at relatively high temperatures and/or under high electric fields, leading to resistance degradation and fatal capacitor failures.
This raises an important fundamental question of how to enhance or suppress migration of oxygen vacancies in perovskite oxides. Two examples will be discussed in this presentation:
1) How to achieve high oxide ion conductivity, high structural stability, and high CO2 tolerance in perovskite oxides by tuning bulk and surface chemistry.
2) How to suppress simultaneously both oxide ion conductivity and electronic conductivity in perovskite oxide BaTiO3 for high-temperature, high-voltage multilayer ceramic capacitors for next-generation high power electronics applications.
St.James-O1
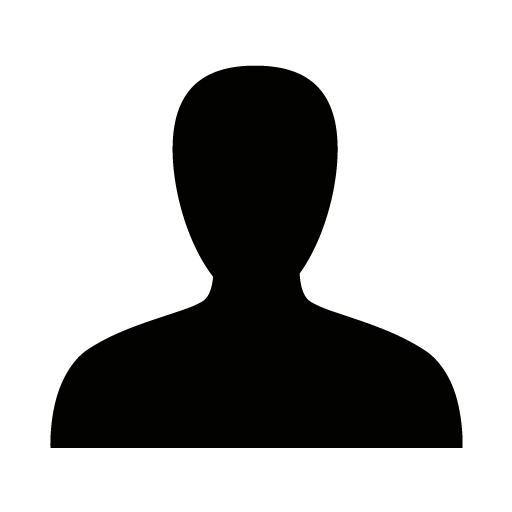
The excessive use of fossil fuels causes global warming, prompting increased focus on eco-friendly energy sources. Among these alternatives, renewable energy sources such as solar and wind power face limitations, as they cannot continuously generate electricity depending on weather conditions. Hence, there is a need for energy conversion technologies capable of storing surplus energy to ensure a stable electricity supply. One promising solution is the reversible fuel cells (RFCs), utilizing electrochemical processes to generate electricity and produce hydrogen within a single device through a reversible reaction [1]. Particularly, reversible protonic ceramic cells (RPCCs) are gaining attention among RFCs, because RPCCs exhibit high power density and efficiency for hydrogen generation, allowing operation at lower and intermediate temperatures. However, due to the lower operating temperature of RPCCs, the kinetics of the oxygen reduction reaction (ORR) and oxygen evolution reaction (OER) are sluggish. Additionally, since RPCCs generate water in the air-electrode, the air-electrode material must exhibit high phase stability in a humidified atmosphere, and fast triple conductivity of oxygen ions, electrons, and protons is required [2].
Recently, numerous studies have focused on enhancing the air-electrode characteristics of RPCCs by doping alkali metals (Li+, Na+, and K+) with alkaline-earth metals having a +2 oxidation state. Doping alkali metals generates oxygen vacancies or electron holes in the structure to maintain the material's electrical neutrality. These formed oxygen vacancies and electron holes significantly enhance the ORR/OER catalytic activity by increasing the conductivity of oxygen ions, protons, and electrons. Moreover, alkali metals, due to their high basicity, substantially contribute to improving the proton conductivity of the air-electrode material through their high reactivity with oxygen and water in the air [3]. This study analyzes the electrochemical properties of air-electrode materials doped with alkali metals. By applying these materials to RPCCs, a significant increase in performance is confirmed compared to conventional perovskite materials.
St.James-O2
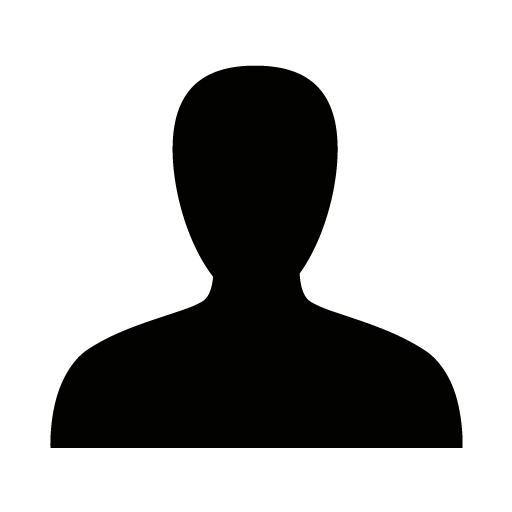
Protonic-ceramic materials, exemplified by BaCe0.8-xZrxY0.1Yb0.1O3-δ (BCZYYb), can facilitate efficient H2O electrolysis between 500 and 700 °C when integrated with effective H2O-splitting electrocatalysis [1]. Several studies have demonstrated that Ruddlesden-Popper oxides like Pr2NiO4+δ (PNO) are efficient water-splitting electrocatalysts [2–4]. PNO conducts both electrons and oxygen ions and can also incorporate protonic defects (OH●) into the crystal structure, which makes it a triple H+/O2−/e− conductor and effective for H2O-splitting [4]. In the range of operating temperatures, PNO's electronic conductivity remains significantly higher than its proton and oxygen-ion conductivities, which limit the region for water-splitting charge transfer reactions near PNO/electrolyte interfaces and increase positrode overpotentials. Collaborative research with West Virginia University has introduced the concept of conformal BaZr0.7Y0.3-yPryO3-δ(BZYP) overlayers on PNO positrode to enhance performance by increasing surface conductivity and consequently, the active surface area. To explore the impact of BZYP overlayers on PNO electrode performance and surface chemistry, BCZYYb electrolyte-supported cells were developed using thin-film PNO positrodes with and without BZYP overlayers via pulsed laser deposition. The thin-film electrodes enabled in operando environmental X-ray photoelectron spectroscopy (XPS) to observe surface chemistry of water-splitting on PNO positrodes.
Electrochemical characterization of pre-hydrated cells has been conducted from 550 to 700°C at a positrode H2O partial pressure up to 0.44 bar and negatrode flow 5% H2/Argon. Open circuit potentials (OCP) measured of 0.925 V exhibited 1.2% deviation from thermodynamic value of 0.937 V at 550°C, which increased to 3.6% at 700°C where measued OCP is 0.843 V and thermodynamic is 0.875 V, attributable to increased electronic leakage. V-I polarization curves and electrochemical impedance spectroscopy (EIS) have shown that BZYP overlayers decreased the total area-specific resistance of PNO positrodes by approximately 50% across the range of operating temperatures. EIS data suggested that BZYP overlayers substantially reduced the bulk resistance, which aligns with the hypothesis that BZYP enhances surface conductivity and electrochemically active areas of the electrodes. However, BZYP overlayers did not significantly reduce polarization resistance.
Environmental XPS at elevated temperatures up to 500 °C in 1.5 mbar H2O pressure on thin-film PNO (uncoated and BZYP-coated) electrodes were performed with and without positive bias in a single-chamber in a Scienta-Omicron HiPP Lab system. The Pr4+/Pr3+ ratio for BZYP surface is higher compared to the PNO surface in biased cell condition by 35%. Monitoring surface ratios of Pr4+/Pr3+ and Ni3+/ Ni2+ enables the evaluation of surface kinetics in response to temperature variations and applied bias. This analysis will offer insights into the water-splitting processes on PNO and BZYP coated electrodes.
St.James-O3
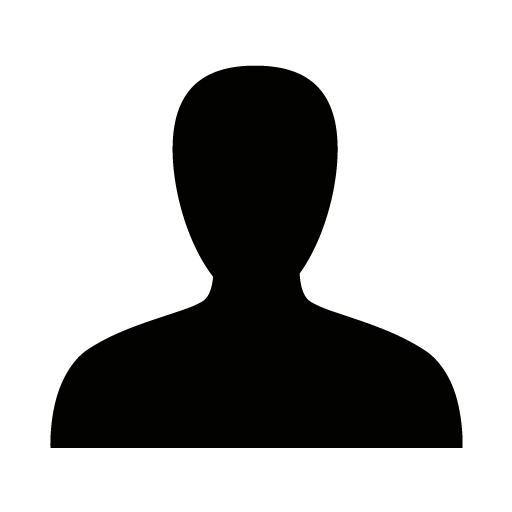
Ba-Zr-based proton conductor is expected as an electrolyte material for protonic ceramic fuel cells (PCFCs). Electron-hole conduction in the proton conductor emerging in oxidizing atmospheres should be addressed to improve power generation efficiency. For that purpose, the nature of protons and electron holes, which are believed to be very similar in electrolyte materials, should be clarified in detail by experimental observation in addition to computational methods. One of the challenges for the experimental observation of charged carriers in the proton conductor is to prepare a fully oxidized sample with only electron holes, unlike a fully hydrated sample with protons, which can be easily obtained by heat treatment in a humidified atmosphere.[1] Therefore, this study focuses on preparing fully oxidizing Ba-Zr-based proton conductors using high pressure with KClO4 as an oxygen source. The candidate material suitable for incorporating electron holes is Sc-doped BaZrO3, which has been suggested to have moderate stability of oxygen vacancies.
BaZr1-xScxO3-δ (BZS; x=0.1-0.5) is prepared by the solid-state reaction method. Protons in samples are removed by drying under a vacuum at 800 °C for 10 h. Electron-hole incorporation was conducted by high-pressure oxidation under 6 GPa for 12 h. ESR and NMR were used to confirm sample oxidation and estimate hole concentration.
1H NMR confirmed the existence of no proton in the oxidized BZS samples; meanwhile, ESR indicated the presence of non-pair electrons only in the oxidized BZS samples. This suggests that hole incorporation into the BZS samples was successful by high-pressure oxidation. 45Sc NMR spectra also supported the full oxidation of the samples and hole incorporation; that is to say, a peak attributed to ScO5 decreased, and a new peak attributed to an electron-hole-coordinated Sc environment emerged in the oxidized BZS samples. This implies that oxygen vacancies are filled, and the high-pressure oxidation incorporates electron holes. The hole concentration in the oxidized BZS samples was estimated to be 9.6, 15.5, and 44.0% for 10%Sc, 20%Sc, and 50%Sc-doped samples, respectively, which reached almost stoichiometric maximum values, meaning full oxidation. The differences and similarities of local structures of fully hydrated and oxidized BZS will also be discussed.
Moore-I1
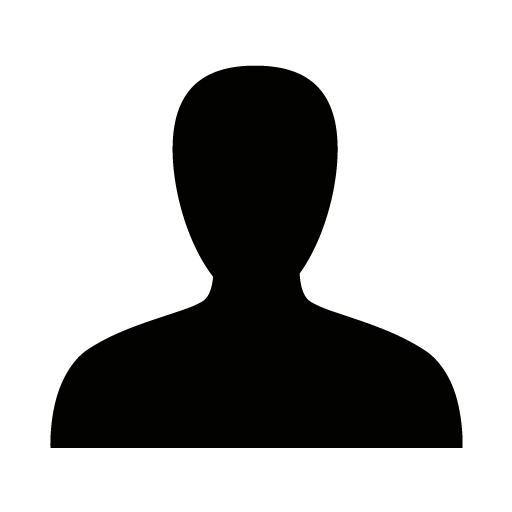
Phase-field models of the Cahn-Hilliard type (the "Poisson-Cahn" framework) have proven useful for models of electrochemical solid-state interfaces due to their fidelity to microscopic experimental datasets and strong connections to first principles calculations. However, kinetic versions of the theory require the solution of a system of fourth-order initial-boundary value differential equations, which is cumbersome even for skilled computational researchers. This talk will present two main results applying Poisson-Cahn to experimental datasets. In one application, a Bayesian kinetic Poisson-Cahn analysis of Scanning Kelvin Probe microscopy on specially-constructed thin film ceria cells performing electrochemical reduction of carbon dioxide yielded estimates of relevant reaction equilibria, rate constants and gradient energy coefficients. The analysis featured an implementation of the Poisson-Cahn in Idaho National Laboratory's MOOSE framework, which implements solvers and provides a convenient user interface. Relevant and customizable codes, including codes implementing the samplers for Bayesian analysis, are available as free software. In another application, equilibrium Poisson-Cahn models applied to TEM-based Electron Energy Loss Spectroscopy measurements at grain boundaries in gadolinia-doped ceria yielded grain boundary conductivity estimates. In this study a "transformed target" statistical approach enabled easy Gaussian process regression to estimate inhomogeneous excess free energies (including gradient effects). The talk will demonstrate how a simple modification of the publicly available FoKL-GP routines enabled the computationally facile estimation of physical quantities directly from microscope data without the aid of large scale computing resources.
Moore-O1
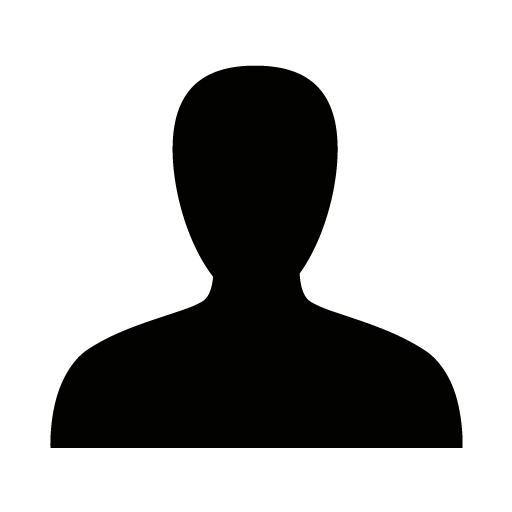
Grain boundary (GB) mass transport, and GB chemistry exert a pronounced influence on both the performance and stability of electrodes for solid oxide cells used in sustainable energy conversion and storage devices. Previous oxygen isotope exchange for the state-of-the-art lanthanum strontium cobalt ferrite (LSCF6428) has revealed a significant enhancement of 4 orders of magnitude in the oxygen diffusivity along the grain boundary when compared to the bulk [1]. In this study, (LSCF6428) was again used as a model mixed ionic and electronic conducting (MIEC) oxide to investigate GB transport at both single and multi-grain length scales. Dense LSCF6428 samples were prepared by sintering pressed pellets at 1250°C and the enhanced grain boundary diffusion was demonstrated by isotope exchange experiments at 350 and 500°C. Atom probe analysis of the exchanged samples revealed distinct changes in the grain boundary composition in the chemically modified layer (CML) of two of the GBs including a decrease in the oxygen content by ~4 %. Thus the enhancement of oxygen diffusivity is attributed to the accumulation of oxygen vacancies at the GB region, but not necessarily at the GB core. In addition to this oxygen deficiency, there were accompanying changes in the cation composition. The boundaries were found to be enriched in Sr and have a matching deficiency in La. The B-site cations, Co and Fe, were found to be enriched in the GB region. This result differs from an earlier study of cation distributions at GBs in La0.8Sr0.2MnO3 by EELS [2] where the B-site cation was found to be depleted and both A-site cations enriched. It is noteworthy that, despite the general trends that can be observed, when studying several grain boundaries (GBs) in LSCF, varying levels of enrichment and deficiency were observed for each cation, emphasizing the differing chemistry of each GB. In addition to the host cations, strong Na segregation was detected at all the GBs examined, despite the low (ppm) level of this impurity in the bulk. The presence of such impurities could affect the space charge potential, subsequently influencing the composition evolution at the grain boundary, as reflected by the distribution of oxygen vacancies [3]. Finally, the study also demonstrates the difference between the crystallographic defined GB width (δ), of ≤ 1 nm, and chemically defined δ, of several nm. This discrepancy could introduce inaccuracies in the calculation of the GB diffusivity (Dgb) and the space charge potential (Φ0) of GBs.
Moore-O2
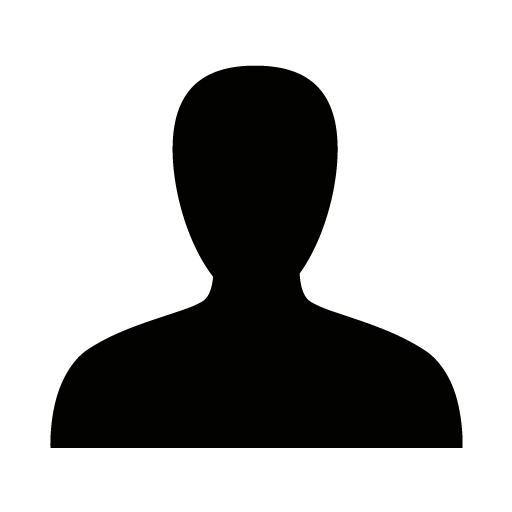
Current state-of-the-art devices for energy conversion and storage still lack the efficiency required for overcoming the energy transition, which is one of society’s major challenges. Polycrystalline thin films with high electronic and/or ionic conductivity play a key role in many energy devices. To tailor the electrical properties of the thin films to the needs of their application, careful material selection and optimized microstructure are necessary. Charge transport properties in new promising mixed conducting materials are typically characterized by impedance spectroscopy (IS). A small sinusoidal excitation signal is applied in the form of a current or a voltage, and the response, which is also sinusoidal, is measured. The frequency range will cover several orders of magnitude making it possible to distinguish between individual transport processes in the spectrum if their characteristic frequencies differ. Additionally the required basic measurement setup is relatively simple. This combination makes IS one of the most powerful and frequently used experimental tools in the field of solid-state ionics.Analyzing impedance spectra is usually accomplished by fitting the experimental data with the impedance of an equivalent circuit consisting of a few components only. This yields macroscopic transport quantities, such as resistances, capacitances and inductances, which are then qualitatively correlated to microscopic transport processes in the sample using theoretical models. The widely used model, the brick layer model, approximates polycrystalline thin films by a network of identical cubes based on the average grain size with a linear potential gradient. However, this model does not consider the impact of different transport paths, among other factors. The true effect of the microstructure on the impedance is still not well understood.
Our study demonstrates the significant influence of microstructure on the impedance of the sample. We perform IS on polycrystalline ceria microstructures using microcontacts as electrodes. Measurements are taken at varying oxygen partial pressures and temperatures. To minimize any artifacts, a specially-built IS measurement setup is used. The microstructures, which are characterized using optical and scanning electron microscopy, have an extension of 20 x 50 µm² with grain sizes of several µm and provide a defined transport path network. Micro Raman spectroscopy is utilized to yield a spatially resolved material characterization. Computer-aided simulations based on an impedance network model are used to analyze the experimental IS data. The simulations accurately map and implement the ceria microstructure. The results indicate that the microstructure has a stronger influence on the impedance than previously thought. Existing models often oversimplify this influence, leading to incorrect assumptions of the microscopic properties. Further investigations are promising for establishing a new correlation model.
Abbey-I1
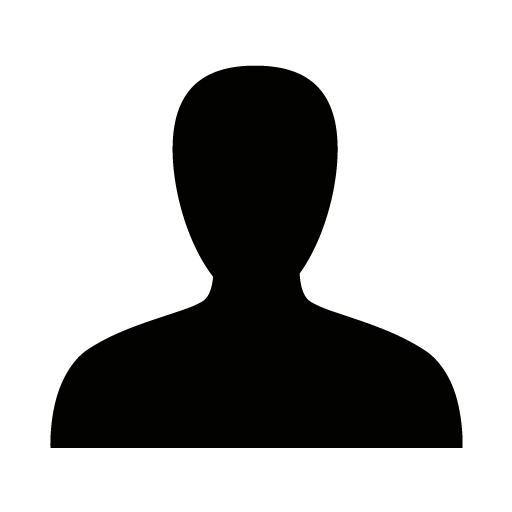
Solid-state batteries stand out as one of the most promising energy storage technologies, holding the potential to revolutionize electric vehicles due to their remarkable attributes, such as high energy density and inherent safety. [1] Despite significant research efforts, the successful implementation of solid-state batteries is still hindered by obstacles such as Li-dendrites, loss of interfacial contact between materials due to electrode volume changes, and chemical incompatibilities between components. [2]
To overcome the current challenges, a deep understanding of the degradation processes taking place during battery operation is necessary. However, studying solid-state batteries in operando on relevant systems and conditions remains challenging, with the main complexity arising from the need to apply high pressures.
In this talk, I will showcase our recent progress in conducting in-situ/operando studies on solid-state batteries. For instance, I will demonstrate how we (i) employed operando cross-sectional nano X-ray diffraction with a spatial resolution down to 30 nm to provide a novel understanding of the formation and propagation of Li dendrites in garnet-type solid electrolytes [3,4], (ii) utilized spatially resolved operando cross-sectional high-energy XRD with a spatial resolution of < 10 mm to examine the phase and stress evolution in solid-state Na-S batteries, and (iii) explored the phase evolution in solid-state Li-FeF3 batteries via in-situ XRD/XANES
Abbey-I2
Yue Qi is the Joan Wernig Sorensen Professor of Engineering at Brown University. She received her Ph.D. from Caltech in 2001. She spent the next 12 years working at General Motors and transitioned back to academia in 2013 as a faculty member at Michigan State University before joining Brown in 2020. She develops multi-scale simulation methods, starting from quantum mechanics, to design materials and interfaces for energy efficiency and sustainability. She has served in multiple inaugural roles aimed at increasing diversity in Engineering.
Rapid filament growth of lithium is limiting the commercialization of solid-state lithium metal anode batteries. Recent work demonstrates that lithium filaments grow into pre-existing or nascent cracks in the solid electrolyte, suggesting that increasing the fracture toughness of the solid electrolytes will inhibit filament penetration. It has been suggested that introducing residual compressive stresses at the surface of the solid electrolyte can provide this additional fracture toughness. [1] One of the ways to induce these residual compressive stresses is by exchanging lithium ions (Li+) with larger isovalent ions such as Na+, Ag+, K+. Here, we first present a multiscale modeling approach to predict the precursors for the ion-exchange process and optimize the macroscopic compressive stress due to the ion-exchange species, concentration, and depth. Due to the complexity of solid electrolyte lattices, the first step is to evaluate the favorable lattice sites for the ion-exchanged dopant ions with density functional theory (DFT) calculations. The diffusion coefficient is then computed as a function of temperature and pressure for different dopant concentrations. The volume expansion coefficient is also predicted by DFT calculations. Based on these predicted parameters, a coupled diffusion-induced stress continuum model is constructed to predict the concentration profile of the exchanged ions (i-ion), based on the diffusion coefficient of i-ion and the chemical expansion coefficient values obtained from both DFT-based calculations. [2] Among the isovalent dopants, the Ag ion-exchanged lithium lanthanum zirconium oxide (LLZO) is shown to induce compressive stress and improve the critical current density (CCD) in symmetric Li cells. How to induce residual compressive stresses while balancing the Li-ion diffusion tradeoff will be discussed.
Abbey-O1
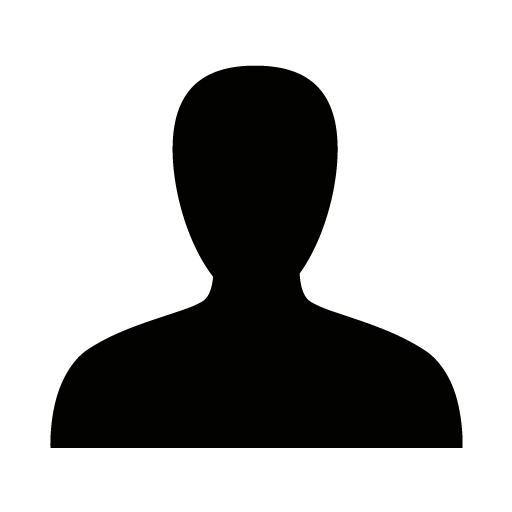
Solid electrolyte-based Li-ion batteries can enable energy storage devices with high energy and power densities due to their compatibility with high voltage cathodes (>5 V vs Li/Li+) and Li metal anode [1]. Among numerous solid electrolytes, doped Lithium Lanthanum Zirconium Oxide (LLZO) has a high room temperature ionic conductivity (≈ 10-3 mScm-1) and suitable electrochemical stability [2]. LLZO when cycled with Li metal in a symmetrical cell configuration (Li-LLZO-Li), continuous stripping and plating results in the formation of Li metal filaments (dendrites) which nucleate on the cathode, propagate through the solid electrolyte, and short-circuit the cell. Broadly, two mechanisms have been proposed to explain these observations, internal pressure build-up due to plating in sub-surface pores and cracks were proposed to result in fracture of solid electrolyte and dendrite growth [3, 4]; the second mechanism postulates metallic lithium deposition inside solid electrolyte due to inherent electronic conductivity of a solid electrolyte [5, 6]. Although, both models offer some insights into possible reasons for dendrites in solid electrolytes, quantitative understanding of dendrite formation that is consistent with high strength of thin lithium metal (with high aspect ratio) is still lacking and are a subject of this work. [7]
Critical current density (ICCD) in LLZO estimated through commonly used cyclic protocols were found not to be a material or system parameter but dependent on the choice of protocol parameters even for samples with similar interfacial resistances and microstructures. Unidirectional plating experiments will be shown to be a cleaner way of estimating ICCD and were found to result in consistently higher ICCDs. More importantly, even pressures as high as 10 and 25 MPa during cyclic experiments were found not enough to recover unidirectional ICCDs. Next, to understand the origin of ICCD in solid electrolytes, a thermodynamically consistent electro-chemo-mechanical model capturing various non-equilibrium process involved in dendrite nucleation was developed. The energy change associated with Lithium ion (Li+) transfer from solid electrolyte to Li metal (Li0) in a prefilled surface flaw was found to be sufficient to break open a hard ceramic solid electrolyte and thus create a pathway for Li0 to plate inside the crack. A quantitative match without any scaling factors between the experimentally observed and theoretically predicted ICCD was observed. Interestingly, ICCD was found to be a range (even for samples with same interface resistance, test protocol and microstructure) rather than a single value due to the inherent geometry of the cells especially at low interface resistances (< 20 Ωcm2). Furthermore, through a combination of experiments and quantitative modelling, possible methods to increase ICCD in solid electrolytes will be described.
Abbey-O2
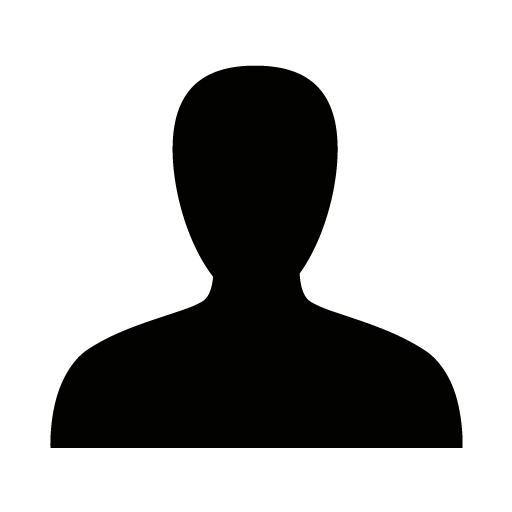
Significant efforts have been made to improve the energy and power characteristics of cathodes in Li-ion batteries, while the research into cathode self-discharge has lagged behind. Self-discharge is the voltage drop experienced by all rechargeable batteries while stored in the charged state. The voltage drop becomes more problematic with increased cathode voltage and temperature. The physicochemical nature of internal reactions leading to cathode self-discharge is hardly understood and seldom discussed.
To tackle this challenge, the structure and redox evolution of commercial LiNi0.5Mn0.3Co0.2O2 electrodes and single crystal cathode thin films upon the self-discharge in carbonate-based electrolyte is revealed by surface-sensitive X-ray scattering, spectrometric and electrochemical characterizations in conjunction with thermodynamic analysis. As evidenced by the interfacial toolkits and theoretical calculations, there is an evolution and growth of cathode surface reduction layer in LP57 electrolyte after self-discharged from different potentials. Structural and chemical chacterizations as well as the elemental depth profiles within cathode thin film confirms proton-insertion-induced layered cathode hydrogenation. Calculation shows that such process is both thermodynamically and kinetically favorable and is triggered by the interfacial hydrogen atom abstraction of methylene group in carbonate solvent on delithiated cathode surface. A combination of experimental and theoretical studies reveals the carbonate-mediated cathode hydrogenation mechanism accounting for the voltage drop in the self-discharge. This offers additional understanding regarding defect generation and the degradation mechanism in layered cathodes beyond the traditional behaviors of lithium-diffusion-induced self-discharge and rock-salt phase induced cathode degradation. This study offers foundational knowledge of the interfacial degradation of cathode that can be translated to the rational design of improved cathodes and electrolytes, and the self-discharge mechanism studies in other electrochemical ion insertion materials and devices.
Gielgud-I1
Fast ionic conductivity is a key requirement for solid-state electrolyte (SSE) in solid-state batteries. There has been growing interest in halide-based SSEs in the AyMX6 (A = Li or Na, M = transition metal or rare earth, X=Cl, Br or I) family, for both Li and Na solid-state batteries. Since the initial study by Asano et al., [1] in which fast ionic conductivity was demonstrated in Li3YCl6 and Li3YBr6 materials, numerous studies have focused on halides materials with hexagonal (HCP) or cubic (CCP) closed packed anion lattices, in which the metal, M, cation is replaced with other transition metal or rare earth elements. [2] Chloride based systems have been shown to give the highest stability against high voltage cathodes, but typically demonstrate lower ionic conductivities than bromide or iodide systems. Although numerous chloride systems have been studied, achieving ionic conductivities > 3 mS/cm at room temperature appears to be a significant challenge. In contrast, Ag and Cu conducting halides based on the RbAg4I5 structure such as RbCu4Cl3I2 have long been known to exhibit exceptional conductivities > 300 mS/cm at room temperature, falling into the class of ‘advanced superionic conductors’.[3,4] An understanding of how rapid ion transport originates in halide materials is fundamentally lacking, which is holding back the design and optimization of future chloride-based advanced superionic conductors.
In this work, we explore the role that A-site cations play on the ionic conductivity of four isostructural chloride SSE systems, A2ZrCl6 where A=Li, Na, Cu and Ag. All four structures adopt the HCP structure when synthesised mechanochemically, allowing for a direct comparison of the impact of the A-site cation. Cu2ZrCl6 and Ag2ZrCl6 display exceptional ionic conductivities (> 3 mS/cm) that are 1–3 orders of magnitude higher than their Li and Na analogues. Using state-of-the-art DFT calculations coupled with transition state searching (TSS), a diverse range of previously unknown ionic conduction mechanisms in the A2ZrCl6 systems are captured.
We demonstrate a new universal transition state model using simple binary halide structures (LiCl, NaCl, CuCl and AgCl) to show that the activation barrier is dominated by the connectivity and relative energies of different octahedral, tetrahedral and trigonal planar coordination at various lattice sizes. We show that an intrinsic ‘lower bound’ activation barrier is always present when there is more than one coordination change along a pathway. We demonstrate that conductivity in the Cu2ZrCl6 system approaches the optimum criterion for the HCP AyMzX6 family of materials. This model is universal to all ionic conductors, including oxide and sulfide SSEs. The results strongly suggest that new halide structural families are required to obtain advanced superionic chloride conductors, and we provide guidance about how these materials may be discovered.
Gielgud-O1
Ana Carolina Coutinho Dutra is a PhD student at Newcastle University in Energy Materials. Her project focuses on studying promising novel solid electrolytes for solid-state batteries via computational modelling and, more specifically, explores ionic conductivity, defect properties and ion transport mechanisms in sodium- and lithium-rich anti-perovskites. Her recent publications include investigations in anti-perovskites systems with zero- to three-dimensional structures (Energy Adv., 2023,2, 653) and a comprehensive review on the computational design of anti-perovskite solid electrolytes (J. Phys. Chem. C, 2023, 127, 18256).
Previously, Ana obtained a First-Class Honours BSc Chemistry from the University of Greenwich and a second BSc in Chemistry with Technological Emphasis from the Universidade Federal do Rio de Janeiro (GPA: 82%). Ana spent her Year Abroad (2014) at the University of Liverpool, where she also worked as a Research Intern during the summer.
In 2020, Ana was awarded the Diploma of Academic Dignity from the Universidade Federal do Rio de Janeiro, in recognition of her academic record of excellence. In the same year, she won the 2020 Brazilian EDP University Challenge with a project focused on the democratization of solar energy via sharing and gamification, which was written in collaboration with fellow students from the University of Sao Paulo.
During her PhD, in 2021, she received the Energy Advances Poster Prize from the Royal Society of Chemistry during the #RSCBatteriesPoster Conference. Additionally, in 2022, Ana received the 1st Place #RSCEnergy Prize from the Royal Society of Chemistry for her poster displayed at the #RSCPoster Conference.
Since 2022, Ana has been a volunteer for the Newcastle University Centre for Energy, creating projects that support fellow PhD students. One of her projects included the creation, curation, co-organisation and chairing of the “Energy Sources” webinar series, which has each one of its episodes focused on a different and pertinent energy source.
When not working, Ana enjoys studying foreign languages. Having Portuguese as her native language and fluency in English, she acquired advanced levels in French (C1) and Spanish (C1) and an intermediate level in German (B1).
Face the current climate emergency, solid-state batteries have been attracting significant attention due to a plethora of potential advantages, such as energy density gains, reduced costs, and safety enhancements. [1] In recent years, Li-rich anti-perovskites have stood out as promising solid electrolyte candidates as they combine high ionic conductivity, stability against Li metal anodes and structural versatility. [2],[3]
Here, defect simulations are used to explore the energetics of defect formation in a range of LixOXy (X = Cl or Br; x = 3–6; y = 1–4) anti-perovskites with zero- to three-dimensional structures, in a work we recently published in the Royal Society of Chemistry’s journal Energy Advances (Energy Adv., 2023,2, 653). Defect calculations are conducted utilising the Mott−Littleton approximation. Long molecular dynamics runs are carried out to assess ion transport in these materials at a range of temperatures (200–800 K). The range of defects investigated includes full, Li-halide and Li-O Schottky defects and Li Frenkel defects. Our calculations predict that whereas almost all these materials present Li-halide Schottky defect pairs as dominant native defects, Cl interstitials are the dominant type of intrinsic disorder in Li6OCl4. We find that the formation of the great majority of defect types is energetically more favourable in the LixOCly series compared to the equivalent structures in the LixOBry set, potentially leading to enhanced Li-ion transport in these materials. We also report that the concentrations of halide Frenkel defects in the LixOBry set are lower than expected in the three and two-dimensional structures, based on the LixOCly series findings for their counterparts. Our molecular dynamics simulations reveal the strong connection between Li-ion dynamics and dimensionality in these anti-perovskite materials, where increased Li-ion diffusion and decreased activation energy can be seen as dimensionality is reduced. Density functional theory simulations and machine learning studies are currently ongoing to further assess ion transport and interfaces in these materials.
Gielgud-O2
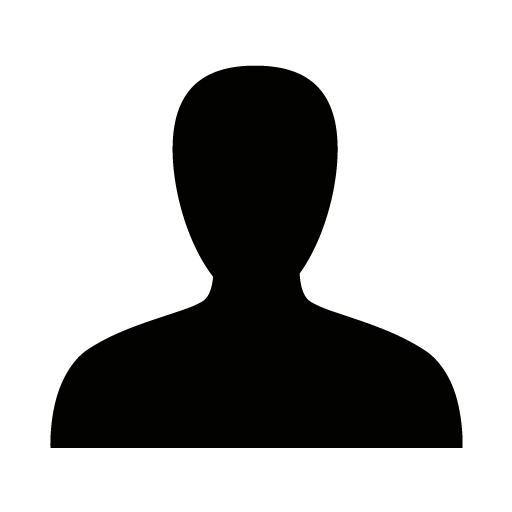
Anode-free lithium metal batteries represent promising candidates for achieving high-energy-density storage capacities. However, their limited cycle life, attributed to the heterogeneous deposition of lithium and the formation of dendrites, poses challenges that must be addressed. The Solid Electrolyte Interface (SEI) plays a crucial role in determining the capacity of these batteries. Consequently, strategically controlling the evolution of the SEI on the anode surface emerges as a key approach to attain desired properties and enhance performance in these devices [1].
Our proposed talk aims to shed light on the understanding of the SEI formation mechanism, with a specific focus on the degradation reactions of the electrolyte. The methodology employed for studying SEI evolution is based on classical molecular dynamics simulation using the REACT package. This approach enables the simulation of large-scale systems and longer periods compared to ab initio simulations [2]. The results can serve as an auxiliary tool for screening and selecting appropriate electrolytes that effectively guide SEI formation towards desired properties.
Furthermore, an atomistic depiction of the SEI significantly contributes to an enhanced understanding of experimental observations. In conclusion, our research aims to make a substantial contribution to the selection of optimal electrolytes for SEI modulation and to improve the capabilities of anode-free batteries.
Gielgud-O3
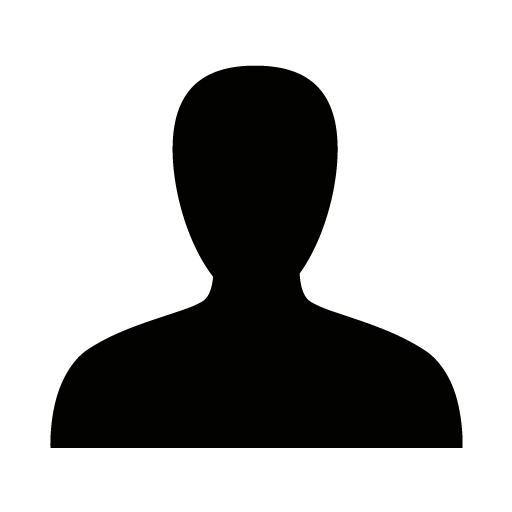
By replacing organic electrolyte solutions with a solid inorganic equivalent, all solid-state batteries (ASSBs) can potentially mitigate the safety issues known for conventional Li-ion batteries, and provide enhanced energy densities when coupled with the Li metal, the ultimate Li-ion anode. Mechanical and (electro)chemical incompatibilities between the ASSB solid components, however, lead to high resistances, curtailing the Li-ion transport at their interfaces.
In this talk, I will introduce a high-throughput modelling approach based on first principles and machine-learning potentials to explore novel ASSB materials with superior features as well as to rationally design more stable interfaces between ASSB components using our automated interface generation code (INTERFACER). I will also elaborate on a specific application that lead to the discovery of a new class of solid Li-P-S electrolytes, Li2S─Li3P solid solutions, which are intrinsically stable electrochemically against the Li metal anodes. I will discuss the mechanisms underlying the higher ionic conductivity and higher electrochemical stability of these new Li-P-S solid solutions as compared to other well-known thiophosphate electrolytes, as revealed by the atomic-level simulations.
Fleming-K1
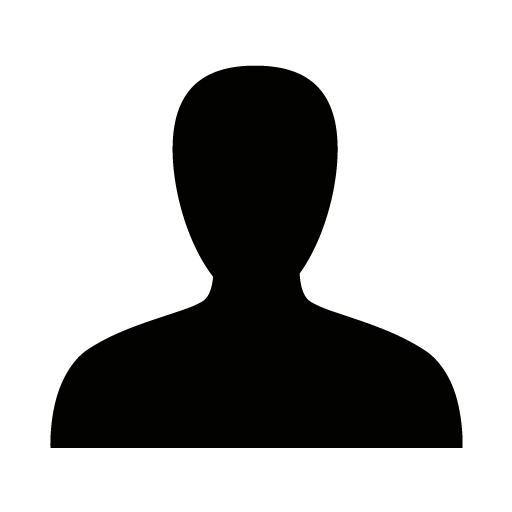
One of the critical degradation issues in Solid Oxide Fuel Cells (SOFCs) is the poisoning by impurities at cathodes [1,2]. The mechanisms of impurity poisoning, especially by Cr and S are reviewed and discussed based on our experimental results as well as literature data. For precise analyses of mass transports at (La,Sr)(Co,Fe)O3/(Gd,Ce)O2/(Y,Zr)O2 interfaces, the microstructures and elemental distributions of elements are examined as well as 18O isotopic oxygen exchange trace diffusion profiles. The motion of Sr in (La,Sr)(Co,Fe)O3 strongly affected the surface oxygen exchange reaction rates by the Sr-segregation at the surface and formed SrCrO4 and SrSO4. The difference between Cr and S poisoning is also discussed given chemical reactivities and resulting chemical compounds as well as electrochemical-induced effects. During the poisoning, rearrangement of phase composition may occur at the reaction products, leading to the formation of Sr-segregated phase, and perovskite phases. Also, some SrCrO4 and SrSO4 phases may evaporate by the existence of water vapors. This rearrangement of phases and side reactions may cause positive effects by eliminating the reaction products and forming 214/113 phase interfaces, where the cathodic reaction can be active. Such recovery reaction from the poisoning is supported by several poisoning experimental results.
The presentation will be made on the overview of such poisoning reactions and recovery reactions given mass transport at the cathode in SOFCs. As shown in Figure, the mass transports and diffusion of elements are discussed.
Fleming-O1
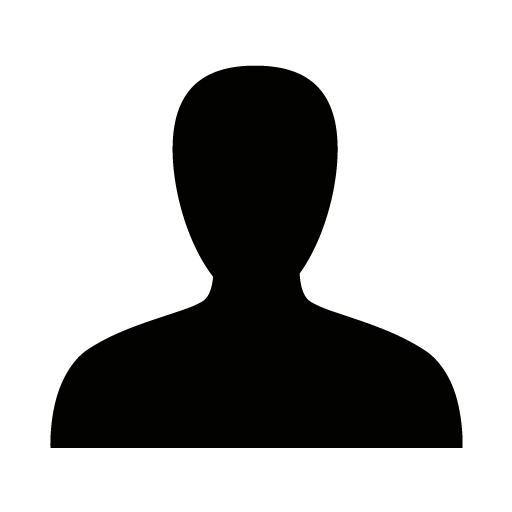
The oxygen reduction in a Solid Oxide Fuel Cell is a complex process that is greatly influenced by the type of material used, its microstructural characteristics, and the operating conditions.
Using Kröger and Vink's notation, the overall reaction can be written as ½O2(g)+2e′+VO..→OOx. This complex reaction involves several steps: diffusion of molecular oxygen, dissociation of molecular oxygen at the electrode surface, diffusion of oxygen or partially ionized atoms at the solid surface or their incorporation into the solid, charge transfer, diffusion of ions into the solid, etc… with different time scales. Whereas gaseous diffusion is a slow process, ionic diffusion in solids is rapid.
Here the calcium cobaltite Ca3Co4O9+δ (CCO), which can be viewed as an emerging electrode for Solid Oxide Fuel Cells or Solid Electrolyser Cells [1-4], was studied as a model SOFC air electrode material. In order to increase the density of triple-phase boundaries between the gas and the two materials, a CCO powder with small grain size was prepared using a citrate route. For screen printed 50/50 wt % CCO (citrate)/CGO composite electrode deposited on a symmetrical cell with a CGO electrolyte, an ASR of 0.35 Ω.cm2 was achieved at 700°C, under air. To go further in the understanding of the oxygen reduction reaction, the cell was then carefully studied at variable temperatures and under variable oxygen partial pressures, combining calculation of the distribution function of relaxation times (DFRT) and complex nonlinear least squares fitting (CNLS) of impedance spectra. This study revealed that this composite is primarily limited by the adsorption dissociation of oxygen on the CCO and CGO surface. Based on this result, the performances of the electrode were improved by partial substitution of calcium with strontium for which better kinetics had been evidenced for oxygen exchange at the surface [5]. A specific surface resistance of 0.15 Ω.cm2 was reached at 700°C.
Fleming-O2
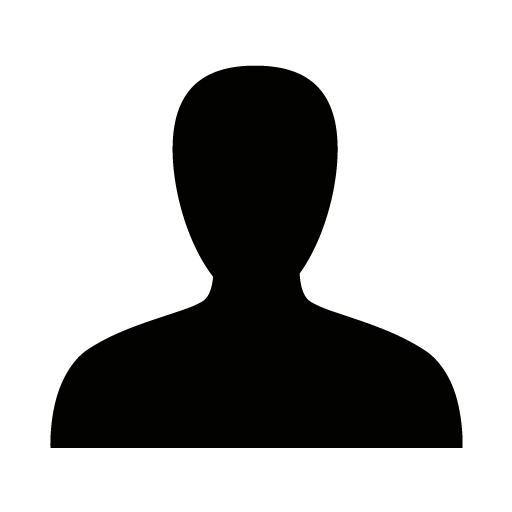
Keywords: Solid Oxide Fuel Cell, electrolysis, electrode, Ba2Co9O14
Among innovative air electrodes for solid oxide cells, the barium cobaltite of formula Ba2Co9O14 (BCO) is promising. It corresponds to the first term of the Ban+1ConO3n+3(Co8O8) series whose structure consists of a stacking of [Co8O8] sandwiched in between [Ba2CoO6] layers. With an electrical conductivity of 100-240 S.cm-1 from 450 to 650°C [1-2], it is competitive to the classical cathode material LSM with sLSM = 50-350 S.cm-1 from 700 to 1000°C [3]. Because of a high thermal expansion coefficient (22.10-6 K), it was studied in composite with Ce0.9Gd0.1O1.95 (CGO). An Area Specific Resistance (ASR) of 0.5 W.cm2 was first reported for a composite containing 30% in weight of CGO [4]. By varying the composition and the electrode thickness of the electrode deposited by screen printing, an ASR of only 0.08 W.cm2 was obtained at 700°C on a symmetric cell made of 50% of BCO and 50% of CGO with a powder prepared by solid state route [5]. These high performances were recently confirmed with an ASR of only 0.3 W.cm2 at 600°C for a composite with Ce0.8Gd0.2O1.9 [6]. Here, to go further in the understanding of the mechanisms involved in the oxygen reduction reaction, a careful study of the electrochemical response and of the performance of this material was carried by impedance spectroscopy combining CNLS-fit and calculation of the distribution function of relaxation time (DRT), varying the experimental conditions: atmosphere, electrode thickness, electrode composition, grain size, interfaces, …
Fleming-O3
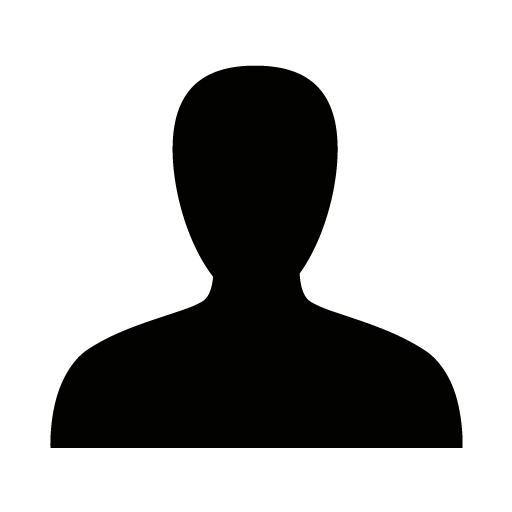
One way to achieve enhancement of the electrochemical performance of solid oxide fuel cell cathode at low temperatures is to mix two oxides with dissimilar structures to form a composite electrode. To understand the enhancement factor of the composite electrode consisting of ionic conducting oxide, Ce0.9Gd0.1O1.95 (GDC), and mixed ionic and electronic conducting oxide, La0.6Sr0.4CoO3-δ (LSC), the electrochemical measurement was performed. It was found that the area-specific conductivity (σe) of the composite electrode enhanced compared to the LSC porous electrode at low temperatures (T < 873 K) under high oxygen partial pressure region (1 – 10-1 bar). The transmission line mode (TLM) was utilized to understand the enhancement factor of the composite electrode. The experimental values for both LSC and composite electrodes (30% LSC: 70% GDC volume ratio) were nearly twice as large as the calculated values at the low temperature. However, the calculated values at 873 K were similar to the experimental values. The discrepancy could be due to the accuracy of the calculated microstructure on the porous electrode, although another possible reason may exist.
In the other case, the enhancement of the ionic conducting pathway due to the existence of the GDC was considered in the calculated σe by TLM for the composite electrode. Considering the low-temperature measurements, the effect of surface diffusion is inevitable. Thus, there are two competing reaction pathways: surface reaction and surface diffusion. The surface diffusion could be one of the possible reasons for the discrepancy between calculated and measured σe since the TLM only considered the surface reaction. Thus, it is necessary to reveal the enhancement factor and reaction mechanism by considering two reaction pathways. Therefore, in this study, the existence of an additional reaction pathway to understand the enhancement factor is discussed.
Fleming-O4
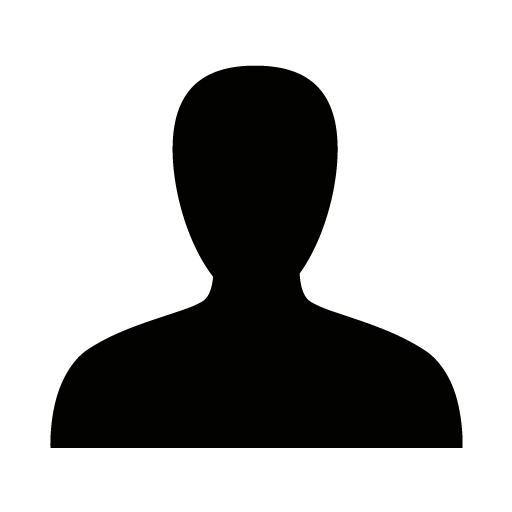
Reversible solid oxide cells (r-SOC) are a key technology for realizing carbon neutrality given their ability to readily switch between fuel cell (FC) and electrolysis cell (EC) modes. Cr-induced degradation of the oxygen exchange kinetics at the air electrode remains one of the main challenges to improving the long-term stability of r-SOC 1. Our research group has previously demonstrated the degradation and recovery of fluorite structured Pr0.1Ce0.9O2 solid oxide fuel cell (SOFC) cathode performance by control of its surface acidity 2-5. Here, we extend the investigation of the applicability of acid/base engineering to (i) the more highly electrically conductive mixed ionic and electronic conducting (MIEC) and popular perovskite structured (expressed as ABO3) La0.6Sr0.4(Co0.2Fe0.8)O3 (LSCF), (ii) at higher operating temperatures, and (iii) in r-SOC operating mode.
The effect of acid/base engineering on LSCF was first evaluated with the aid of symmetric cells operated in air. Cr and Ca-based oxides were used as acidic and basic additives, respectively. Cr infiltration caused a 20-fold increase in the LSCF polarization resistance compared to the unifiltrated mode in the temperature range from 500 °C to 650 °C. However, subsequent Ca infiltration largely recovered the oxygen reduction reaction activity by reducing the polarization resistance to less than twice that of the unifiltrated sample, demonstrating the ability of applying acid/base engineering to LSCF-based cathodes.
However, we discovered that these degradation and recovery effects induced by acidic and basic additives become suppressed at more elevated temperatures between 800 °C and 900 °C. STEM-EDS microstructure observations show that high-temperature conditions drive the dissolution of Cr and Ca based secondary phases into the perovskite structure of LSCF leading to a time dependent loss in their efficacy. Therefore, in order to apply acid/base engineering to the high-temperature regime for LSCF, strategies to control the dissolution of the secondary phase particles are necessary.
We also report on our evaluation of the effect of acid/base engineering on the operation of r-SOC cells. As demonstrated for FC operation in a previous study 3, we were able to demonstrate the degradation in performance with Cr-infiltration and its recovery with Ca-infiltration as well for the oxygen evolution reaction in EC operation. These results suggest that acid/ base engineering can also be applied to reversible cells.
Westminster-K1
There is continued interest in Niobate-based oxides for a variety of energy-related applications, from mixed conducting electrode materials for Li+/Na+ batteries to high energy density dielectrics for pulse power electronic applications. Here we report on the influence of A-site vacancies on the structural stability and electrical functionality of (i) NaNbO3 perovskite and (ii) Sr2NaNb5O15 tetragonal tungsten bronze.
(i) Partial replacement of Na+ by Bi3+ with the creation of A-site vacancies in NaNbO3 produces a solid solution based on Na1-3xBixNbO3 which induces intrinsic clustering of lattice distortions that leads to displacive order–disorder behaviour with increasing x [1]. This remarkable solid solution initially exhibits interesting polar dielectric phenomena such as anti-ferroelectricity (x = 0.00) and relaxor ferroelectricity (x = 0.10) before undergoing an order-disorder transition that promotes co-existence of weakly coupled relaxor ferroelectricity and Na+ ion conduction (x = 0.20). We now compare and contrast this range of functionality with that based on a range of A-site Rare Earth (RE3+ = La-Nd) and Alkaline Earth (AE2+ = Ca,Sr) dopants based on the mechanisms, Na1-3xRExNbO3 and Na1-2xAExNbO3, respectively. This allows a ‘holistic’ view of the electrical properties of A-site deficient NaNbO3 to be presented.
(ii) Sr2NaNb5O15 (SNN) has been reported as a novel high-temperature dielectric material [2]; however, there are questions about its thermodynamic stability and the influence of A-site vacancies based on the existence and thermal heat treatment of the solid solution Sr2+xNa1-2xNb5O15 with 0 ≤ x ≤ 0.20. Here we present results on the thermal stability and dielectric properties of a solid solution based on x = 0.20 and designed as Sr2.2Na0.6+yNb5-yTiyO15 with 0 ≤ y ≤ 0.20 to systematically vary the A-site vacancy concentration from ~ 6.66 to 0%. The results show the thermodynamic stability is linked to the concentration of A-site vacancies and that the lower permittivity maximum at ~ -70 oC for x = 0.20 is invariant with the level of A-site vacancies; however, the magnitude and temperature of the ferro- to para-electric transition at ~ 230 oC for x = 0.20 show substantial variations. These results are discussed in a wider context on how to control the thermodynamic stability and temperature coefficient of capacitance in SNN-based dielectrics.
Westminster-O1
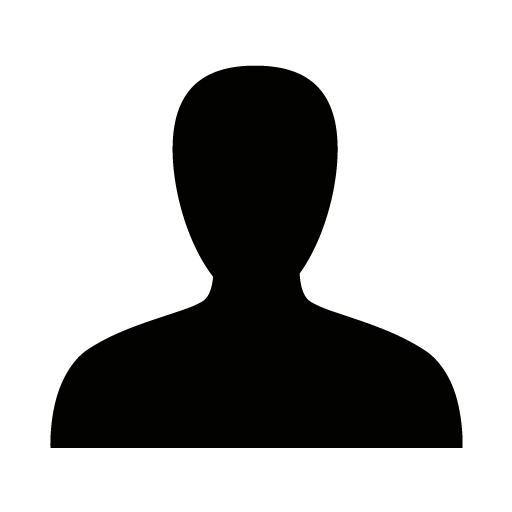
Lithium titanate, Li4Ti5O12 (LTO), due to its zero-strain behavior during cycling, excellent chemical stability and cyclability, has been a promising anode material for solid-state batteries (SSB) applications. By restricting the material into two dimensions, as a thin film, the range of applications broadens further for traditional battery materials into integrated circuits, sensors, batteries for flexible and internet of things devices, and memristors1-4. In these applications, a careful control of the mixed Li+ ionic – electronic carriers and their dynamics requires attention to alter either for an optimal battery storage anode or in a memristive application, operating at different cycle, life spans and voltages. In the past, improvements in electron conduction and lithium ion (Li+) diffusion have been achieved by the inclusion of dopants in powder instances of LTO5-8. Dopants such as Ta5+, Co3+, Mn4+, can change the electronic conductivity by altering the electron concentration or the ionic diffusivity by increased lattice sizes or affecting Li+ pathways. However, dopant studies completed on thin films are still limited. To bridge this gap in research, we investigate the effect of solid solution dopants on LTO thin film’s kinetics and how they impact functionality in device applications, specifically Li+ driven thin films for energy storage in batteries and information storage in memristors. The dopants chosen for this study were Nb5+, V5+, which replace the Ti4+, Mg2+, for Li+, and Cu2+, which substitutes both Li+ and Ti4+. Films were first produced using pulsed laser deposition (PLD) using doped LTO targets. To characterize these changes in our electrochemical kinetics, we measure X-ray photoelectron spectroscopy (XPS) and electrochemical impedance spectroscopy (EIS) observing variations in the atomic valences and conductivity and diffusion. Next, we characterize how the kinetic changes affect electrochemical performance in two applications, thin-film batteries and memristors. From our memristors, we see a trend among the hysteresis curves where Ti-replacing dopants, Nb5+ and V5+, have a decrease in hysteresis while the Li-replacing dopants, Mg2+ and Cu2+ see an increase in hysteresis and symmetry. In our battery samples, cyclic voltammetry measurements demonstrate the shifting of the cathodic peaks during lithiation shift to lower voltages, relating to the changes in material kinetics. Collectively, the insights of these measurements and others completed in this study contribute fundamentally to the understanding of the kinetics of Li movement in LTO, and how it might impact our thin-film applications, memristors and batteries.
Westminster-O2
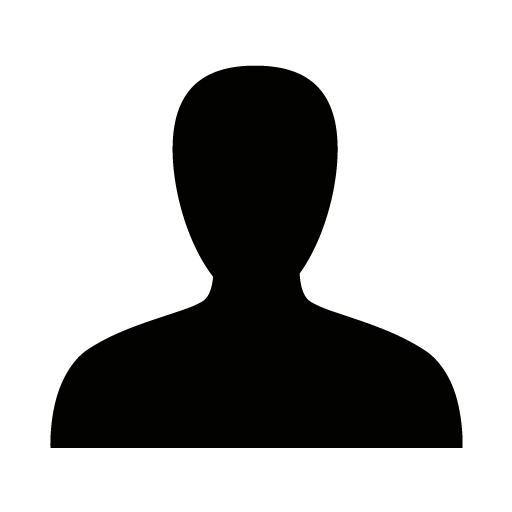
Fran's work focuses on emerging materials for optoelectronics and optoionics applications. This work includes thin film growth by pulsed laser deposition, materials characterization, and photodevice application.
Neuromorphic computing and memory devices using memristive effects have the potential to contribute fast and non-binary computing beyond transistors. Lithium based oxides like lithium titanate (i.e., spinel Li4Ti5O12) provide ample opportunities to switch resistively due to the strong electronic state-lithiation coupling and multiple phases existent beyond their typical use in battery anodes.[1,2] Recently, it was demonstrated that above band gap illumination could lead to enhancement of ionic transport in doped binary oxides by modulating space charge barriers at grain boundaries.[3] In this work we employ photoconductive Atomic Force Microscopy (phAFM) and observe photocurrent generation, consistent with the presence of space charge layers at interfaces in lithium titanate thin films. Phototitration measurement at varied bias voltage reveals the existence of a memristive effect, and that the current is much more rapidly generated upon switching under the 375 nm light illumination. Furthermore, we test the memristive switching characteristics between dark and under illumination for cross-bar measurements up to 200 kV/cm. The memristive device shows a noticeable difference under the dark vs. light; the photomemristive behavior shows a gradual increase of current with time with a concomitant decrease in device resistance. Collectively, we demonstrate an opto-ionic effect for lithium titanate films allowing for memristive operation with a wider range of read/write options for next-generation opto-memristor devices.
Westminster-O3
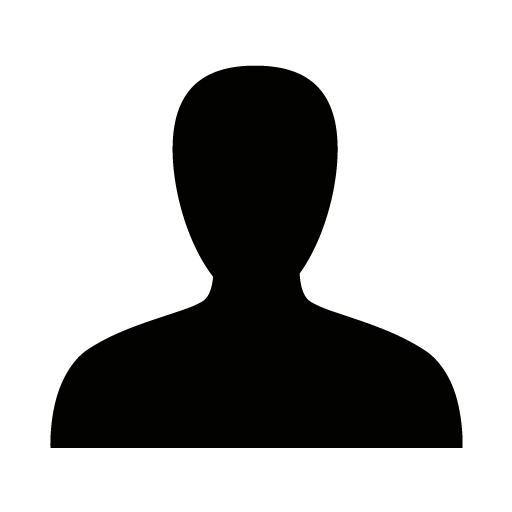
While machine learning is rapidly revolutionizing countless disciplines, training neural networks requires large memory and consumes enormous amounts of power. Thus, fundamentally new computing paradigms are needed to greatly improve the energy efficiency of computing. Electrochemical random-access memory (ECRAM) is an emerging technology for energy-efficient neuromorphic computing. ECRAM devices allow for low-energy, analog tuning of a channel resistance by electrochemically controlled ion insertion (extraction) into (from) the channel material, which is typically a semiconducting metal oxide. To preserve electroneutrality, cation insertion usually leads to changing electronic charge carrier concentrations in the bulk, and it is generally assumed that this change is at the origin of the resistance modulation in ECRAM devices. However, there is evidence in the literature that the resistance of polycrystalline WO3, a common channel material for ECRAM devices, can be dominated not by the bulk resistivity, but by grain boundary resistance[1], which is associated with space charge effects resulting in the depletion of electron carriers in the vicinity of the interface. We use electrochemical impedance spectroscopy (EIS) combined with careful choice of source/drain electrode geometry to distinguish between bulk and space charge resistances such as those arising at grain boundaries or electrode/WO3 interfaces in WO3 channel films. EIS of the channel in a Mg-ECRAM device during programming reveals that in the low ion concentration regime, modulation of space charge resistance dominates, while for higher ion concentration levels, bulk doping is the more prominent effect. We will discuss possible mechanisms for this modulation of space charge resistance, including changing charge carrier concentrations in the space charge region upon ion intercalation and direct reduction of the interfacial core charge density. This novel understanding of additional resistance modulation mechanisms in Mg-ECRAM opens new pathways for exploration, such as the role, if any, that space charge modulation plays when H+ is used as the working ion. These results also call for an informed design of channel microstructure and geometry in ECRAM devices, which is required to aid in meeting the demanding speed and energy efficiency requirements for neuromorphic hardware.
Westminster-O4
The rare earth nickelates have received renewed attention due to the discovery of superconductivity in infinite layered structures under substitutional doping[1] and the observation of widely tunable electronic behavior in perovskite structures for use in analog memory devices[2]. Recent work has demonstrated that interstitial dopants (H, alkali metals) can be introduced into nickelates to change the room temperature resistance by 106 -108. However, the evolution of the bond disproportionation transition as a function of interstitial dopants has not been reported and the doping fraction leading to rich correlated electronic behavior is often unknown. Therefore, the electronic phase diagram in nickelate compounds as a function of interstitial doping is of interest. Here, we carried out lithium doping of PrNiO3 using a dynamic electrochemical process. We constructed electrochemical cells using epitaxial thin films as electrodes and then insert lithium using an electrolyte. For LixPrNiO3, we find that increased lithium doping interrupts bond disproportionation causing a reduction in the ground state resistivity at small fractions 0<x<0.25 with a successively smaller ON/OFF ratio. At larger fractions x>0.25 we observe the disproportionation transition to be destroyed and fully insulating type behavior is observed over T= 5-300K. Raman spectroscopy reveals that lithium introduces structural changes that affect A1g modes which are a sensitive probe of bond disproportionation. Density functional theory calculations confirm the disruption to bond disproportionation with an initial reduction in the bandgap at small fractions and an increase at larger fractions. The results point to interstitial doping as a powerful method to synthesize new phases in strongly correlated systems.
St.James-K1
Proton-conducting oxide cells have promising applications in hydrogen production through steam electrolysis and fuel cells, operating at lower temperatures than those utilizing conventional oxide ion conductors like YSZ. However, proton-conducting oxides exhibit hole conduction in oxidizing atmospheres, leading to electronic leakage in cells, resulting in lower Faradaic efficiency in steam electrolysis and excessive fuel consumption in fuel cells.
While using proton conductors devoid of hole conduction could offer a fundamental solution to these challenges, it proves difficult due to the underlying principles of proton conductivity.
This study investigates methods to mitigate electronic leakage, particularly by controlling mass transport of species that participate in electrode reactions. For instance, enhancing the Faraday efficiency of steam electrolysis was achieved by incorporating Gd-doped ceria as an electron-blocking layer in the air/steam electrode, as depicted in the figure.
Interestingly, experimental findings confirm that such electrode structures with inserted electron-blocking layers do not effectively suppress electronic leakage in fuel cells. While steam electrolysis and fuel cells are often portrayed as operating in reverse, this does not imply precisely opposite processes occur in electrode reactions or electronic leakage reactions.
This presentation will delve into how electronic leakage can be controlled precisely by determining where and how to intervene in steam electrolysis and fuel cells.
St.James-O1
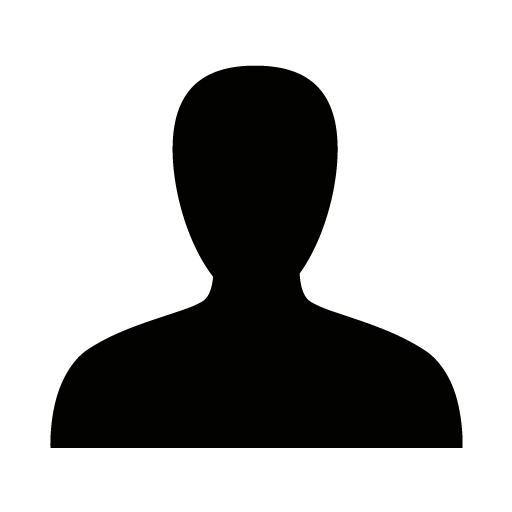
Hydride ion (H–) conductors have made remarkable progress in recent years; in particular, the fluorite-type LaH3–δ series exhibits high conductivity around room temperature. However, its intrinsic character of hydrogen non-stoichiometry still makes its application as a solid electrolyte challenging, for which high electronic insulation is essential. Here, Sr-substituted LaH3–δ with slight O2– incorporation, represented as La1–xSrxH3–x–2yOy (0.1 ≤ x ≤ 0.6, y ≤ 0.171), is synthesized, which exhibits H– conductivity of 10–4 - 10–5 S cm–1 at room temperature. The galvanostatic discharge reaction using an all-solid-state cell composed of Ti|La1-xSrxH3-x-2yOy|LaH3–δ shows that the Ti electrode is completely hydrogenated to TiH2 for x ≥ 0.2, whereas a short circuit occurs for x = 0.1. These experimental observations, together with calculation studies on the density of states and the defect formation energy, provide clear evidence that electropositive cation, such as Sr, doping critically suppresses the electron conduction in LaH3–δ. Achieving a superior H– conducting solid electrolyte is a novel milestone in the development of electrochemical devices that utilize its strong reducing ability (Eº(H–/H2) = –2.25 V vs. SHE), such as batteries with high energy density and electrolysis/fuel cells with high efficiency.
St.James-O2
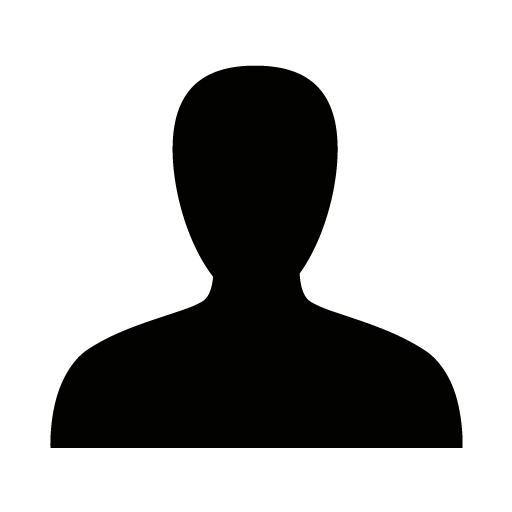
Proton transport in oxides can occur through the material's bulk when a sufficient bulk proton concentration is present, for example by dissociative hydration of oxygen vacancies (e.g. in well-established proton conductors such as Y-doped BaZrO3). On the other hand, surface proton conductivity in adsorbed water layers is expected for a much larger range of materials, in particular at temperatures below ≈300°C. Water-oxide interaction and surface protonic conductivity have been investigated on several ceramic materials, see e.g. [1], [2]. Similar effects are anticipated also for mixed conducting oxides, and may be harnessed for porous electrodes of low temperature protonic ceramic fuel cells. Protonic carriers are expected to generate from water dissociation, followed by transport along the surface water layers and participation in the electrode reactions.
While preceding work focused on surface protonics of electronic insulators, the present study employs the Mg(Al1-xFex)2O4 spinel solid solution (without oxygen vacancies) and praseodymium doped ceria Ce1-xPrxO2-δ (with oxygen vacancies, but nevertheless negligible bulk hydration) as model materials to investigate the interplay of water adsorption, surface protonics and bulk electronic transport. Samples were synthesized by nitrate pyrolysis, and compacted by spark plasma sintering to pellets with relative densities of 50-90%. The water uptake is measured by thermogravimetry at different temperatures and pH2O. Regimes of chemisorption (extending up to ≈600°C) and physisorption (significant below ≈150°C) can be identified, and modelled by mass action laws. Both Fe3+ and Pr4+ substitution affect the surface properties of the synthesized materials, which changes the enthalpy and the concentration of active sites for water molecular adsorption and dissociation.
Conductivity is investigated by impedance spectroscopy in an extended temperature and pH2O range (up to 400 mbar). It depends sensitively on sample morphology (surface area and porosity). In humid gas, all porous samples show a characteristic upturn of conductivity below ≈250°C, which is attributed to surface proton conduction. This can increase the conductivity by more than 8 orders of magnitude relative to dry conditions. The increase of conductivity with the amount of adsorbed water is very steep. This emphasizes the important role of physisorbed water layers atop the chemisorbed layer, which facilitate water dissociation as well as proton mobility. For Mg(Al1-xFex)2O4 as well as for Ce1-xPrxO2-δ the surface proton conductivity is almost independent of the concentration of redox-active Fe or Pr cations and thus the bulk electronic conductivity. This is in strong contrast to the bulk proton transport of mixed conducting perovskites such as Ba(Zr,Y,Fe)O3-d, for which the proton concentration and conductivity decreases significantly already for small amounts of redox-active iron ions [3].
St.James-O3
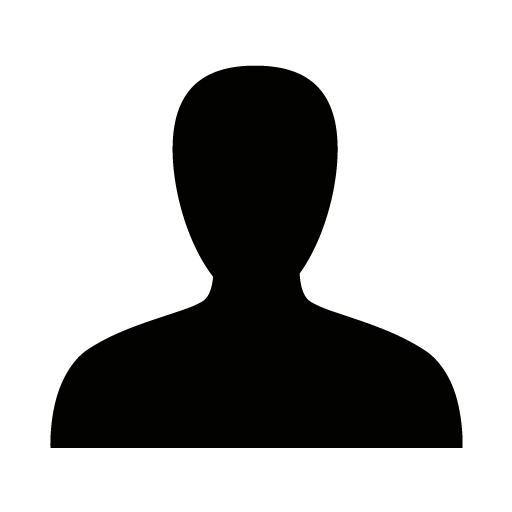
Proton conducting fuel cells (PCFC) are recently among the most promising strategies for tackling the key issue of Solid Oxide Fuel Cells (SOFC) development, namely their high operating temperatures. Their advantage lies mainly in the superior values of protonic conductivity at the low-temperature range, compared to the oxygen ions. However, unveiling the full potential of PCFC technology is currently limited by the performance of air electrode materials. Firstly, the catalytically active zone is often restricted, when only a mixed oxygen ion–electron conductor (MIEC) is applied. This constraint is overcome by the development of triple, proton-oxygen-electron conductors, which allow for the transport of all involved species across the volume of the electrode, thus extending the active region to the whole surface area. Yet, their design is still burdened with multiple challenges involving competition between their transport properties, electrochemical activity, stability, and functional properties. While Ba-based systems are well-recognized for their protonic conductivity, they tend to suffer from several stability-related issues, such as being prone to CO2 interactions, Ba diffusion, or transformation to hexagonal phases. Similarly, incorporating transition metals, especially Co, meant to introduce the electronic contribution to conductivity and enhance electrochemical performance, deteriorates protonic conductivity, and adversely increases TEC values, compromising the functional properties.
Herein, we address these challenges and propose modifications of B-site lattice configuration in the established perovskite triple conductor BaCo0.4Fe0.4Zr0.1Y0.1O3-δ (BCFZY), aimed at enhancement of proton uptake and catalytic activity. We present three new perovskite-structured triple-conducting materials: BaCo0.4Fe0.4Zr0.1Zn0.1O3-δ, BaCo0.4Fe0.4Ce0.1Y0.1O3-δ, BaCo0.4Fe0.4Ce0.1Zn0.1O3-δ, synthesized by the modified Pechini method. The proposed modifications result in an increase in oxygen deficiency, which may be correlated with improved catalytic activity. By comparative studies under dry and humidified atmosphere, we confirm the presence of triple conductivity in the studied systems. The results for modified systems demonstrate enhanced stability of protonic defects and indicate higher proton uptake. Moreover, the changes in total conductivity under varied atmospheres further allow us to identify the mechanism of protonic defect formation. Additional analysis, including measurements of the Seebeck coefficient, attempts to explore the modifications’ influence on the defect structure, as particularly the Ce-substitution exhibits recognizable impact, despite involving only 10% of the B-site sublattice. As we verify by dilatometric measurements and in-situ high-temperature XRD, the improvements of transport properties come without deterioration of the thermomechanical behavior. The chemical stability is tested against both PCFC and O-SOFC electrolytes. Finally, by EIS measurements, we study how the observed enhancements are reflected in the electrochemical performance as PCFC electrodes.
Overall, our analysis indicates the three newly proposed triple-conductors as viable candidates for PCFC air electrodes and illustrates an effective approach to the design of a new generation of cathode materials.
St.James-O4
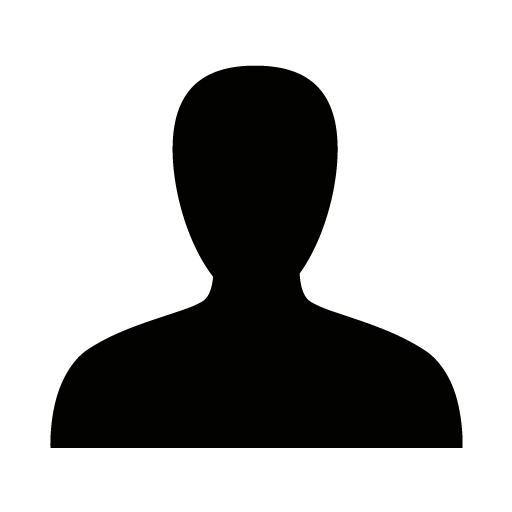
Mixed protonic and electronic conductors are promising materials for electrodes in hydrogen-related electrochemical devices and are essential for the realization of high-performance fuel cells, electrolysis cells, and hydrogen separation membranes. Materials exhibiting oxide ionic conduction in addition to protonic and electronic conduction termed as the triple-conducting oxides recently attract much attention as a proton-conducting mixed conductor; however, their working temperature is limited at >600 °C. Because the operating temperatures of fuel cells and electrolysis cells are expected to be lower, extending into the intermediate temperature range of <500 °C, it is necessary to explore new mixed protonic and electronic conductors that work by a different mechanism than conventional ones. Hydrogen is known to dissolve in n-type oxide semiconductors and behave as a donor according to the following reaction: H2 → 2Hi・+ 2e’. The protons generated by this reaction are expected to be highly mobile because their countercharges are not immobile point defects but electrons that are delocalized throughout the crystal. Here, the dissolution of hydrogen into the Nb-doped TiO2 crystal and its mixed protonic and electronic conductivity in H2 were investigated.
Total electrical conductivity that corresponds to the partial electronic conductivity varied in response to the atmosphere (air or H2) and was larger in H2 than in air. It was over 1 Scm−1 at above 200 °C in H2. The electron carrier density in air was 1.3×1018 cm−3 and increased to 1.3×1019 cm−3 after annealing in H2. Thermal desorption spectrometry showed the H2 and H2O gas emissions only from the sample annealed in H2. The amount of H2 and H2O released was converted to the proton densities in the Nb-doped TiO2 of 2.8×1019 and 3.5×1020 cm−3, respectively. Since the increase in electron carrier density and the amount of H2 released after H2 annealing were almost the same, the increase in total electrical conductivity in H2 is due to the increase in electron carrier density resulting from the dissolution of hydrogen and its ionization according to the above reaction. The reason why protons released as H2O do not generate electron carriers is not yet clear. The partial protonic conductivity was determined by dc and ac impedance methods using a proton-conducting glass electrolyte as an electron-blocking electrode. It varied from 2×10−4 Scm−1 at 200 °C to 1×10−3 Scm−1 at 250 °C with an activation energy of 0.74 eV. Its partial protonic conductivity of 1×10−3 Scm−1 at 250 °C is only an order of magnitude smaller than that of BaZrO3-based electrolyte at ~600 °C, which is extremely high despite the low temperature. Assuming that all dissolved protons with a density of 3.8×1020 cm−3 contribute to the protonic conductivity, their diffusion coefficient was calculated to be 7×10−7 cm2s−1 at 250 °C, which is larger than the previously reported 1×10−8 cm2s−1 for pure TiO2 [1]. This may be because the electron carriers introduced by the Nb donor spread throughout the crystal, facilitating proton transport.
The result that Nb-doped TiO2 exhibits high protonic and electronic conductivity at around 250 °C in H2 strongly suggests that n-type oxide semiconductors dissolving hydrogen could be a new field to explore mixed protonic and electronic conductors. Furthermore, this finding has great potential for extending the operating temperatures of hydrogen-related electrochemical devices to lower temperatures.
Moore-K1
Ion-conducting metal oxides are ubiquitous in various electrochemical energy conversion applications, offering high conversion efficiencies and environmental stability. The ionic transport properties of polycrystalline electrolytes used in practical electrochemical devices, however, can be dominated by the internal grain boundary (GB) interfaces. The blocking of ionic transport at GBs has typically been assigned to local space charge barriers that form due to the segregation of lattice defects or impurities to the grain boundaries, resulting in the development of net charge at these interfaces. These space charges induce mobile ion defect depletion zones adjacent to the GBs that can decrease the overall ionic conductivity by orders of magnitude and increase their corresponding activation energies. While this space charge phenomenon associated with GBs in solid electrolytes has been well known and characterized for some time, little effort to date has been made to demonstrate the ability to engineer these space charge barriers by control of the GB chemistry and/or structure. In recent years, our research team has demonstrated the ability to modulate ionic conduction in polycrystalline Gd doped ceria (GDC) solid electrolytes by illumination with above bandgap light[i]. The electrons and holes thus generated are separated by the built-in space charge fields localized at the GBs, leading to a net decrease in barrier height and thereby an increased effective ionic conductivity. In an attempt to optimize this opto-ionic effect, we have investigated means for controlling the built-in GB space charge barrier heights.
Pursuing an approach that we first demonstrated in Pulse Laser Deposited (PLD) ceria thin films in 2009,[ii] GDC films with 3 at % Gd were grown on single crystal substrates that served as sources of selective cation up-diffusion along the GDC GBs, taking advantage of the many orders of magnitude higher diffusivities of cations along oxide GBs compared to those in the grains. MgO and Al2O3 substrates were selected with the expectation based on the difference in ionic radius of cations from Ce that while Mg would substitute on Ce GB sites, leading to localized negatively charged species, Al would enter interstitially as a localized positively charged species. Given that such GDC materials, as grown, exhibit net positive GB charge,[iii] we expected that Mg in-diffused films would show decreased GB barrier heights while Al in-diffused films would show increased GB barrier heights. Indeed, we demonstrated this capability, dropping barrier heights to as low as 0.01 eV for Mg in-diffused films while increasing barrier heights to as high as 0.43 eV for Al in-diffused films. In this report, we review the in-diffusion experiments, discuss the derived Mg and Al GB diffusivities as determined from secondary ion mass spectrometry (SIMS) measurements and subsequent profile analysis, and briefly discuss the implication this may have for detecting illumination sources.
Moore-O1
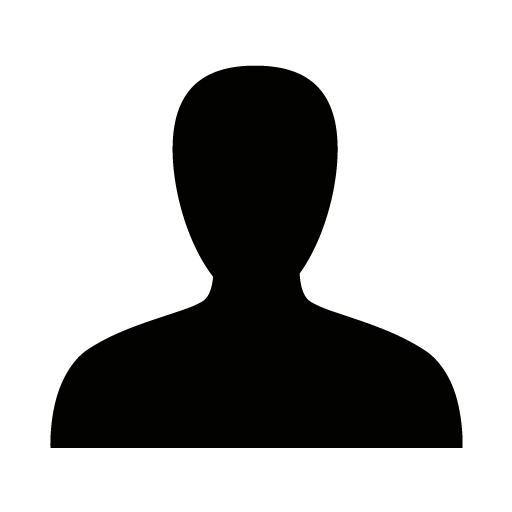
In all-solid-state batteries, key issues are ion transport at grain boundaries and the interface between the active material and electrolyte. It is necessary to identify the dominant ion transport mechanism that inhibits ion conduction in batteries. In this study, we visualize Li-ion diffusion at grain boundaries via secondary ion mass spectrometry (SIMS) at −110 °C using an isotope exchange technique for perovskite-type Li0.29La0.57TiO3 (LLTO) as a model solid electrolyte.[1] This technique enables to evaluate the grain boundary diffusion coefficient.
LLTO was prepared via isotope exchange at 22 °C for 59 h and introduced into the SIMS system immediately after 6Li exchange. The SIMS image clearly reveals that the relative 6Li fraction C changes rapidly at the grain boundaries via comparison with the laser microscope image taken from the same position. This result means that the grain boundary resistance is the main factor increasing the total resistance of LLTO solid electrolytes. Considering the continuity of the diffusion flux across the interface between the bulk and the grain boundary, we can calculate the grain boundary diffusion coefficient Dgb from 6Li concentration gradient in the bulk nearby the boundary dC/dy|bulk, the difference in the 6Li concentration at the grain boundary dCgb and the bulk diffusion coefficient Dbulk. Based on the line profile from SIMS image, the derivative coefficient dC/dy|bulk is determined as 1.1 cm−1 using a quadratic function, and dCgb is 0.02. If the Dbulk of 2.6 × 10−8 cm2 s−1 measured by PFG-NMR [2] is used, we obtain Dgb/l = 1.5 × 10−6 cm s−1. Assuming the typical grain boundary thickness l = 0.5 nm [3], then Dgb = 7.6 × 10−14 cm2 s−1, and the calculated Dgb is five orders of magnitude lower than Dbulk.
Moore-O2
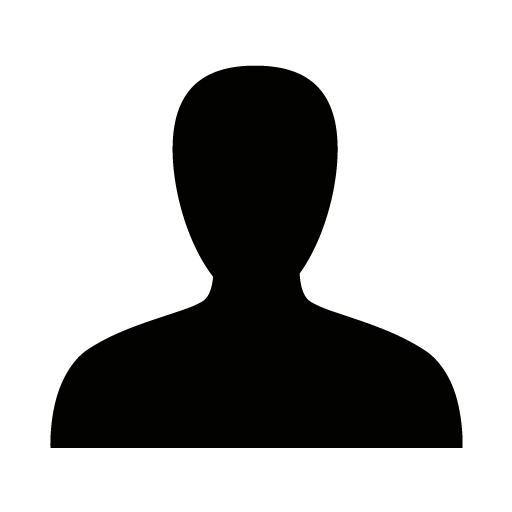
All-solid-state batteries (ASSBs) are candidates for the next-generation of battery electric vehicles. They potentially enable the use of lithium metal as an anode material thereby highly improving the energy density of the battery. Among the most promising candidates for solid electrolytes are polycrystalline materials such as LLZO, due to their high ionic conductivity and chemical stability [1].
The understanding of internal grain boundary interfaces is of interest both to optimize performance and to possibly prevent the formation of dendrites [2]. Improved understanding of solid electrolyte-solid electrolyte interfaces informs the design of hybrid electrolyte systems.
One often used tool in battery analysis is Electrochemical Impedance Spectroscopy (EIS). By utilizing an inverse Fourier transform on the truncated interface impedance, we calculate a minimal capacity value from the experimental data as an additional informative parameter. The truncated part is where overlap and uncertainties render the experimental data unreliable.
Using our continuum scale model, we simulate impedances for a range of possible interface configurations both for one interface and for a 3D polycrystalline structure. Space charge layers and their effects are included in the model and material properties are informed by atomistic simulations.
In our contribution we simulate impedances at ASSB interfaces using continuum scale modelling. The comparison to experiment helps us gain a better understanding of the relevant interfaces including the effect that different interface configurations have on the resistance. The gained understanding can inform the development and manufacturing of future ASSBs.
Moore-O3
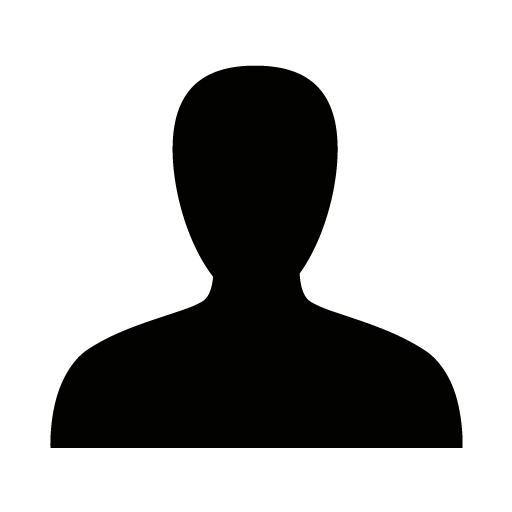
Lithium dendrite formation and insufficient ionic conductivity remain primary concerns for the utilization of solid-state batteries. Given that the interpretation of experimental results for polycrystalline solid electrolytes canbe difficult, computational techniques are invaluable for providing insight at the atomic scale. Here, first-principles calculations are carried out on representative grain boundaries in four important solid electrolytes, namely, an anti-perovskite oxide, Li3OCl, and its hydrated counterpart, Li2OHCl, a thiophosphate, Li3PS4, and a halide, Li3InCl6, to develop the first generally applicable design principles for grain boundaries in solid electrolytes for solid-state batteries. The significantly different impacts that grain boundaries have on electronic structure and transport, ion conductivity and correlated ion dynamics are demonstrated. The results show that even when grain boundaries do not significantly impact ionic conductivity, they can still strongly perturb the electronic structure and contribute to potential lithium dendrite propagation. It is also illustrated, for the first time, how correlated motion, including the so-called paddle-wheel mechanism, can vary substantially at grain boundaries. These findings reveal the dramatically different behavior of solid electrolytes at the microscale compared to the bulk and its potential consequences and benefits for the design of solid-state batteries. These design principles are expected to aid the synthesis and engineering of solid electrolytes at the microscale for preventing dendrite propagation and accelerating ion transport.
Moore-O4
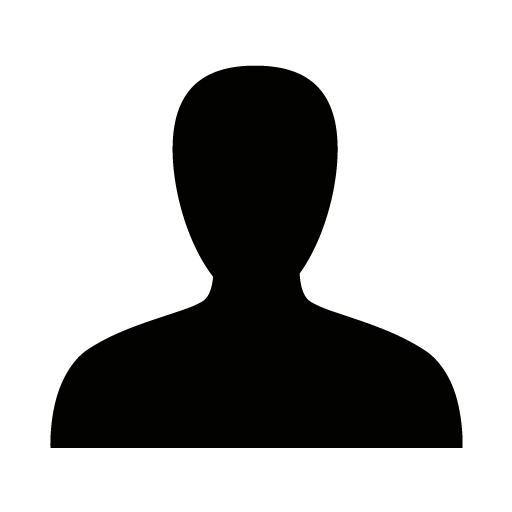
The effects of grain boundaries (GBs) in NaSICON solid electrolytes, with a Na3Zr2Si2PO12 composition, on sodium-ion battery performance are explored in this study. It is revealed that diffusion at GBs is significantly slower than in the bulk, particularly at lower temperatures. Notably, in the direction perpendicular to GB, the diffusivity is found to be much lower, and the barriers for Na-ion transport are shown to reach close to the experimental value.[1] Local structural changes, such as faulty bonding in the Twist case, are also demonstrated by the research to substantially alter Na-ion transport by transforming the shape of the hexagonal bottleneck. This transformation results in a narrower bottleneck diameter, impacting ion movement at GB. Analysis of the electrostatic potential near GBs uncovers varying charge distributions due to these structural changes, with distinct behaviors observed in Twist and Tilt scenarios. These findings enhance our understanding of how atomic-scale interactions in NaSICON solid electrolytes influence sodium-ion battery efficiency.
Abbey-K1
Solid-state batteries based on a ceramic electrolyte and lithium metal anode promise to transform battery safety and energy density, but both charging and discharging rates are limited to below what is required. On discharge, voids form in the lithium metal at the Li/solid electrolyte interface due to limited Li metal creep at practical stack pressures and under practical current densities. These voids accumulate on cycling, leading to detachment of the Li anode and consequently high local currents during charge, triggering the growth of dendrites (filaments of Li metal that penetrate the ceramic electrolyte) resulting in short-circuit and cell failure. Furthermore, even without any prior voiding, charging currents are limited to levels too low for practical applications by dendrite formation. Important efforts are being made to understand dendrites in ceramic electrolytes and to mitigate them.
We describe the formation and progression of lithium dendrites, informed by observing dendritic cracks operando using X-ray computed tomography (XCT). Dendrites are found to follow a two-stage process of initiation then propagation, with distinct mechanisms for each. Initiation occurs in sub-surface pores where pressure builds to exceed the local fracture strength at the grain boundaries, by the mechanism of slow lithium extrusion. Propagation involves dry cracks, with Li driving the crack forward from the rear by a wedge-opening mechanism, rather than lithium at the crack tip, as had often been assumed previously. Informed by the description of dendrite cracks, we control the microstructure of an Argyrodite (Li6PS5Cl) solid electrolyte and examine the impact of different microstructures on the dendrites. These studies consider how microstructure influences critical current densities for dendrite growth.
Abbey-O1
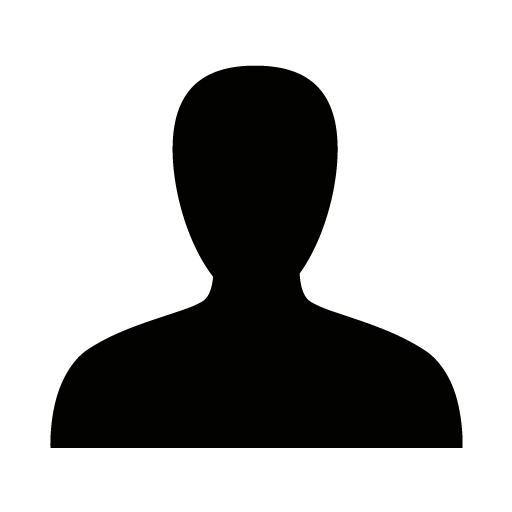
Although solid-state batteries with metal anodes promise to enable safer, higher energy density batteries, metal protrusions (dendrites) grow when charging faster than a critical current density.1,2 It is generally believed that dendrites grow when plating-induced stresses exceed that required for fracture of the solid-electrolyte.2–4 It is commonly assumed that the threshold stress for failure depends on the electrolyte's fracture toughness—commonly taken as a material constant.2–4 Here we use operando birefringence microscopy5 to directly measure dendrite-induced stresses. We find that increasing current densities increase the dendrite velocity. Dendrite-induced stresses appear to evolve with time—even during dendrite propagation—in a fashion that depends on the current density or dendrite velocity. Cryogenic Scanning Transmission Electron Microscopy (Cryo-STEM) reveals decomposed electrolyte phases at the dendrite tip. This decomposition is associated with a volume contraction. All experiments were conducted on the most electrochemically stable Li-ion conducting solid electrolyte (tantalum-doped lithium lanthanum zirconium oxide).6 Together, these experiments allow separate study of electrochemical and mechanical phenomena underlying dendrite growth in ceramic electrolytes.
References
1. Sudworth, J. L., Hames, M. D., Storey, M. A., Azim, M. F. & Tilley, A. R. An analysis and laboratory assessment of Two Sodium Sulfur Cell Designs. Power Sources 4, 1–18 (1972).
2. Sharafi, A., Meyer, H. M., Nanda, J., Wolfenstine, J. & Sakamoto, J. Characterizing the Li–Li7La3Zr2O12 interface stability and kinetics as a function of temperature and current density. J. Power Sources 302, 135–139 (2016).
3. Fincher, C. D. et al. Controlling dendrite propagation in solid-state batteries with engineered stress. Joule 6, 2794-2809 (2022).
4. Porz, L. et al. Mechanism of lithium metal penetration through inorganic solid electrolytes. Adv. Energy Mater. 7, 1701003 (2017).
5. Athanasiou†, C. E., Fincher†, C. D. et al. Operando measurements of dendrite-induced stresses in ceramic electrolytes using photoelasticity. Matter (2023).
Abbey-O2
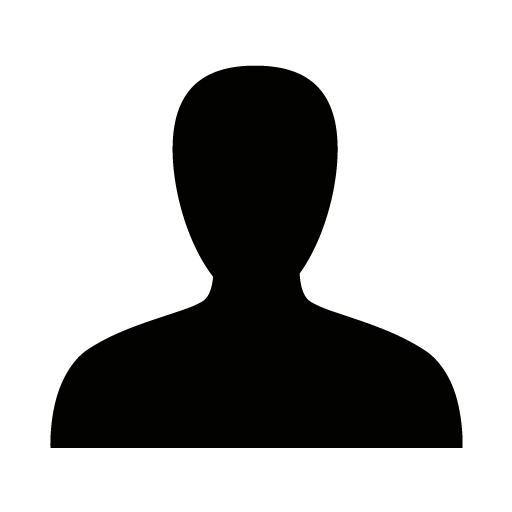
What is the advantage of an all-solid-state battery (ASSB)? The solidification of a battery surely contributed to enhance the safety due to using a non-flammable solid electrolyte; however, the sufficient electrochemical interface is difficult to achieve between solid and solid, unlike between liquid and solid. Thus, the R&D of ASSB always faces on the interfacial issues. Especially in case of oxide-based ASSB, the thermal reaction between an electrode and an electrolyte during co-sintering is also the additional interfacial issue. The thermally reacted interphase formed during co-sintering has been widely studied to optimize the sintering conditions for bulk-type ASSBs with high Li-ion conductive candidates (e.g. perovskite-type conductors, sodium super ionic conductors (NASICONs), and garnet-type conductors). However, the resulting battery performance during operation remains inadequate. Therefore, successful co-sintered ASSBs are currently limited to specific electrode-electrolyte combinations. Previously, we reported the good performance of an oxide-based ASSB due to an impurity-free interface between a layered rock-salt LiMO2 electrode (e.g. LiCoO2) and an LISICON-type oxide solid electrolytes (e.g. LGVO; Li3.5Ge0.5V0.5O4) following co-sintering.1)
In this study, we prepared homogeneous distributed composite texture by actively utilizing the thermodynamic two-phase coexistence stability of aforementioned LiCoO2-LGVO combinations. Heterogeneity between the electrode particle and solid electrolyte within the electrode layer, such as agglomeration of the electrode particle, causes electrochemical reaction distribution, which affects battery characteristics such as the cycle capacity retention of ASSB.2) We exploited the thermodynamic stability of LiCoO2 and LGVO to create finely composite composites of these. By applying heat to the formed precursor to cause recrystallization and grain growth, we created a self-assembled electrode texture in which submicron-sized LiCoO2 particles were relatively uniformly distributed within the LGVO solid electrolyte. We will present on the fabrication method of self-assembled electrode layer, the stability of the LCO/LGVO interface based on thermodynamic calculations, and battery performances such as cycle capacity retention for bulk-type solid-state battery (Au | LiCoO2-LGVO self-assembled electrode layer | LGVO | dry polymer | Li).
Abbey-O3
One of the strategies to improve the performance of the positive electrode in Li-ion batteries consists of mixing multiple active materials, typically two of them. If properly selected, this results in synergistic effects that could potentially enhance the performance beyond what the rule of mixtures predicts. Unfortunately, the internal dynamics of these electrodes remain poorly understood. One synergistic effect involves lithium redistribution within electrode components, especially after periods of high discharge current that force one of the materials to accommodate excess lithium. This interaction has been termed as “buffer effect” and could potentially be exploited to enhance the cell´s performance in high intermittent loads, often imposed to EV batteries.
Blended electrodes containing binary mixtures of four of the most used active materials, namely LiNi0.5Mn0.3Co0.2O2 (NMC), LiMn2O4 (LMO), LiFePO4 (LFP) and LiFe0.35Mn0.65PO4 (LFMP) have been investigated with the aim to understand the contribution of each material as a function of state of charge during both continuous1 and pulsed discharges (3C) . A special experimental decoupled blend electrode setup2 and operando synchrotron XRD and XANES measurements on real blended electrodes are used.
Results derived from both experiment types are in good agreement and shed light to internal processes and their dependence on material’s voltage profile and state of charge (SoC). It is found that individual materials are subjected to rates significantly higher than the cell’s nominal. Furthermore, the magnitude and directionality of the buffer effect strongly vary depending on the binary combination of materials and the battery SoC.
Gielgud-K1
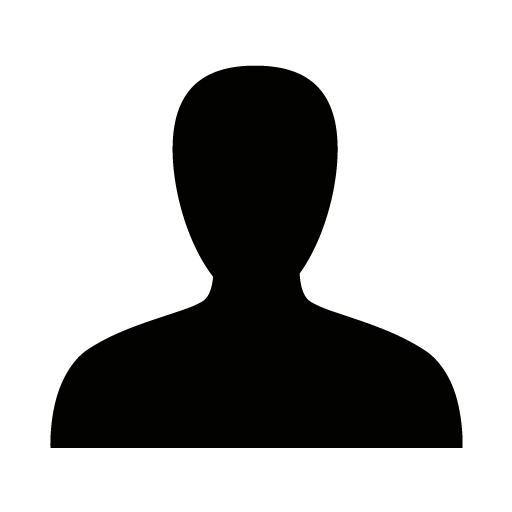
This presentation explores new cobalt-free air electrodes for protonic ceramic cells, focusing on Fe-containing perovskites oxides. We first introduce a Sr0.9Ce0.1Fe0.8Ni0.2O3-δ (SCFN) perovskite-based nanocomposite for air electrodes and Ni-Fe alloy support for fuel electrodes, improving RePCCs' performance [2]. The SCFN nanocomposite, exhibiting electrocatalytic surfaces enriched with CeO2 and NiO nanoparticles, enables superior oxygen exchange and transfer. The produced RePCCs demonstrate excellent power density and stability.
In the second part of the talk [2], we analyze the challenges protonic ceramic fuel cells encounter. In particular, the cobalt-free perovskite, Ba0.875 Fe0.875Zr0.125O3−δ, is developed using ab initio simulations by showing that hydration and oxidation need to be facilitated while ensuring stability. While the former properties are enhanced by Ba-site deficiency, Zr substitution enhances stability. The prepared cell demonstrates superior electrochemical activity, achieving a peak power density of 0.67 W cm−2 at 500 °C. This work presents promising, efficient, and durable strategies for protonic ceramic cells.
Gielgud-O1
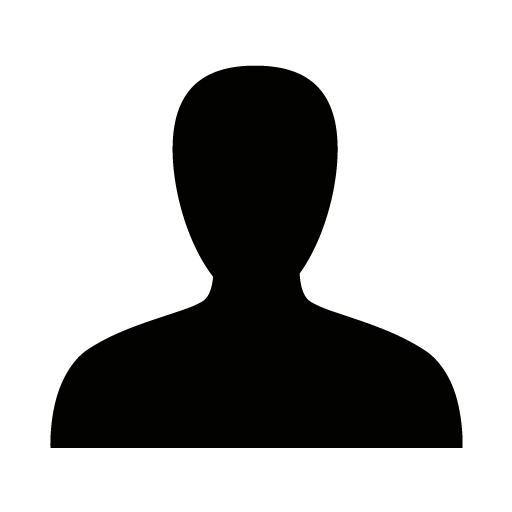
High-throughput computational screening and machine learning offer significant potential for exploring diverse chemical compositions and identifying novel inorganic solids [1,2]. Despite these advancements, identifying high-performance materials with specific properties remains challenging. In response, we propose "Materials Discovery by Interpretation (MDI)," a hybrid approach designed to efficiently develop high-performance materials by leveraging both machine learning and interpretation. We applied MDI to explore fast proton-conducting oxides at an intermediate temperature of 300C. Our machine learning model identifies new host materials for proton conductors, nominating rhombohedral SrSnO3 [1]. In the first attempt, we observed proton conductivity in Sc-doped SrSnO3, which exhibited a relatively high bulk proton conductivity of 0.001 S/cm at 380C. Our interpretation of fast proton conductors focuses on symmetric cubic oxides with high Sc solubilities [3]. Realizing our interpretation in a stannate-host perovskite has led to heavily Sc-doped BaSnO3. Using this methodology, we discovered proton-conducting oxides exhibiting 0.01 S/cm conductivity at 300C and high chemical stability [4].
Gielgud-O2
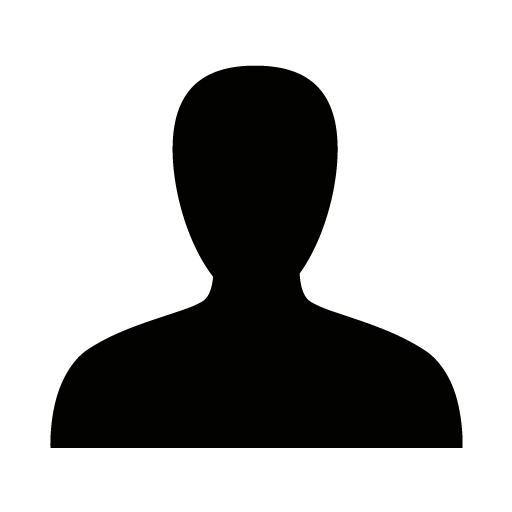
Barium zirconate demonstrates significant potential as a solid electrolyte in ceramic fuel cells due to its elevated protonic conductivity after doping with trivalent oxides like yttria and subsequent hydration. In the partially hydrated material transport of both protons and oxygen ions is possible. The underlying transport mechanisms include single ion jumps of protons and oxygen ions but also the concerted migration of both ions in a OH-vehicle jump. Furthermore, the individual migration steps which constitute the overall conductivity are affected by the local environment of the transition and the defect-defect interactions.
In this study we present the investigation of proton and oxygen ion conductivity in yttrium doped barium zirconate from first principles employing density functional theory, Metropolis Monte Carlo and Kinetic Monte Carlo simulations. Fundamental defect interactions and migration barriers are described on the atomic scale by density functional theory. The thermodynamic properties, in particular the relation between water vapor pressure and hydration degree, are simulated by Metropolis Monte Carlo methods. Finally, the partial conductivities are obtained using Kinetic Monte Carlo simulations. We demonstrate the influence of the defect interactions on the hydration and conductivity of the material and specifically include cooperative migration mechanisms beyond single ion hopping.
Gielgud-O3
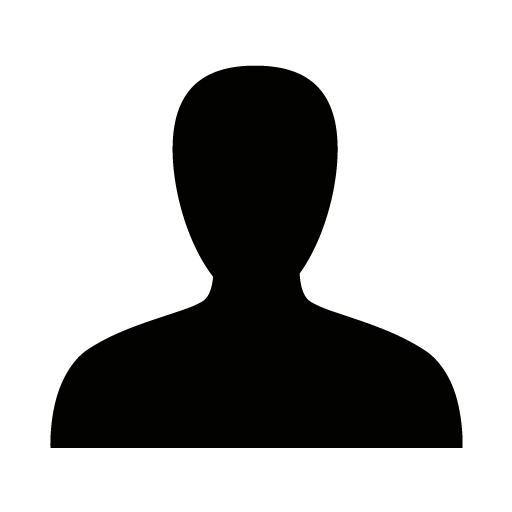
Oxide ion conductors (OICs) currently excel at temperatures exceeding 700 °C. However, this high temperature range presents limitations in material options and energy requirements for ion mobility initiation. To integrate OICs into modern energy storage technologies, it is crucial to identify materials enabling oxygen mobilization at lower temperatures while maintaining thermodynamic stability. This entails reducing the energy needed for the diffusion process and expanding the range of compatible materials.
In this study, we leverage autonomous workflows and Density Functional Theory (DFT) calculations to screen 5,400 A7B4B’O20 hexagonal perovskites. These materials exhibit quasi-2D interstitial oxygen diffusion at the interface. Our computational screening aims to identify systems with energy barriers below 1.0 eV, utilizing the Nudged Elastic Band (NEB) approach.
The culmination of our efforts yielded a promising selection of 37 candidate structures, each meticulously scrutinized and deliberated upon. This research marks a significant step towards overcoming conventional temperature constraints in oxide ion conductor technology, offering potential breakthroughs in energy storage.
Fleming-I1
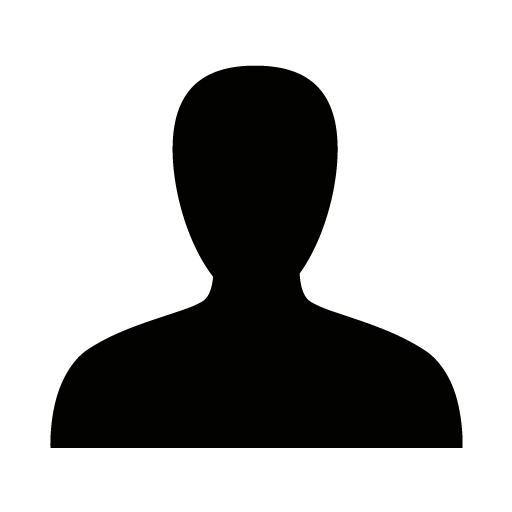
Elisabeth Djurado is a Full Professor of Materials Science at University of Grenoble Alpes (Grenoble INP Phelma), France. She completed her Ph.D. degree in Chemistry from Blaise Pascal University at Clermont-Ferrand, France in 1988 in the domain of fluorides. Throughout her career, she was a Research Scholar at the University of Pennsylvania, US (1989-1990) in the domain of superconductors. Her main research interests in LEPMI (Laboratory of Electrochemistry and Physicochemistry of Materials and Interfaces) Grenoble, France since 1990 include the elaboration and the structural/microstructural and electrochemical investigations of nanostructured ceramics and interfaces for solid-state ionic and especially for solid oxide cells at intermediate temperature. She has published about 128 papers in international peer-reviewed journals (H – Index 31, Web of Science) and she has directed 20 Ph.D. theses.
Solid oxide electrolysis cells (SOECs) operating at ~750-850°C are high-temperature electrochemical energy-conversion devices that efficiently produce green hydrogen. The high operating temperatures of the SOECs combined with the electrode polarizations can cause physical and chemical degradation, reducing the lifetime of the cells. To increase the durability, one can lower the operating temperature to ~700°C. However, this leads to a decrease in the electrochemical performance due to higher activation energy and lower reaction kinetics, especially in the oxygen electrode. The implementation of new materials and microstructures that would improve the electrochemical performance and durability of SOEC remains a major issue. Recently, praseodymium oxide, PrOx, has received attention as an innovative MIEC oxygen electrode for solid oxide fuel cells, known for its very high electrocatalytic activity at low temperatures. However, its relatively poor electronic conductivity does not seem to reduce its electrochemical activity when used as an active functional layer [1].
This work is proposed to study the performance and the stability of PrO1.833 material as a promising oxygen electrode onto a standard solid oxide electrolysis half-cell. For this purpose, a nano-structured PrO1.833 active functional layer (AFL) was coated by electrostatic spray deposition (ESD) following our previous microstructural optimization [1,2]. A hierarchical nanostructured porous columnar-type layer was successfully obtained. The study was performed considering this AFL topped by strontium-doped lanthanum manganite (LSM) as the current collecting layer on a standard half-cell supported, composed of a typical Ni-YSZ cermet, a thin YSZ electrolyte, and a GDC (Ce0.9Gd0.1O2-d) barrier layer. The electrochemical measurements have been investigated before and after a long-term test (at 700 °C for 1000 h) under electrolysis mode (- 0.5 A cm‑², with 90 vol.% H2O and 10 vol.% H2 at the hydrogen side with a total flow rate of 15.5 Nml min-1 cm-2, air at the oxygen side and steam conversion (SC) of 25%). Rather good initial performance and reasonable degradation rate of 5.8 % kh-1 were measured. Impedance spectra recorded before and after the long-term test revealed an increase in the pure ohmic resistance and the contribution at high frequencies. On the other hand, the durability test did not affect the medium and low frequencies in the impedance spectra. SEM observations have shown that a Ni particle coarsening and migration away from the hydrogen electrode/electrolyte interface occurred in operation. This microstructural evolution could explain a significant portion of the evolution of the impedance spectra. Additionally, the structural and elemental evolution of the oxygen electrode through its thickness was thoroughly investigated by synchrotron µ-X-ray diffraction and fluorescence at micro-XAS beamline at Swiss Light Source (SLS), Paul Scherrer Institute (PSI), Switzerland. The degradation of the oxygen electrode was mainly attributed to the phase transitions of PrO1.833 while no substantial elementary interdiffusion was detected between the different layers. Finally, additional structural characterizations were performed on samples annealed at 700 °C for 1000 h and at 800 °C for 700 h without applied current. The results are discussed to provide a better understanding of the stability of the praseodymium oxide [3]. In particular, the structural analyses of the oxygen electrode aged under anodic polarization revealed the presence of GDC and an electrochemically active PrO1.714 phase in the majority along with a small amount of PrO1.5 ≤ x ≤ 1.7 phase at the GDC/AFL interface. To conclude this PrO1.5 ≤ x ≤ 1.7 phase present in the minority in the aged SOEC could be at the origin of the increase in the ohmic resistance since it is expected to be less conductive than PrO1.833.
Fleming-O1
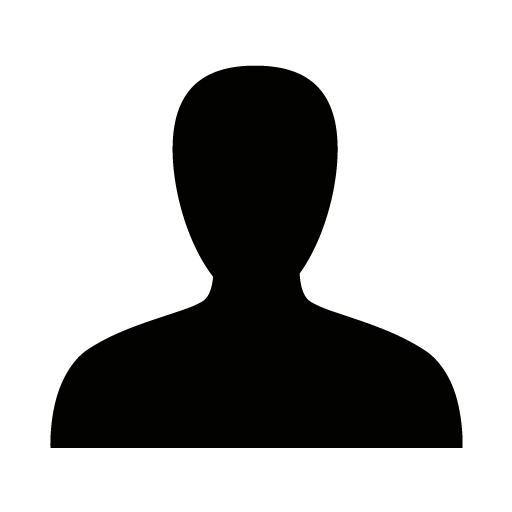
Cobalt-based perovskite oxides (LaxSr1−xCoyFe1−yO3−δ, Ba0.5Sr0.5Co0.8Fe0.2O3−δ, etc.) show excellent electrochemical performance as cathode materials for intermediate temperature solid oxide fuel cells (IT-SOFCs), but it has limited application due to low thermal stability by the easy evaporation and reduction of cobalt [1]. For this reason, cobalt-free cathode materials are being studied, and Ba0.5Sr0.5FeO3-δ (BSF) can have a thermal expansion coefficient (TEC) similar to that of ceria-based electrolyte through doping [2]. BSF has disordered oxygen vacancies and a three-dimensional oxygen diffusion path, which are advantageous for oxygen ion conduction [3].
In our previous study, the thermal stability of BSF was improved by doping Mn into the B-site to reduce the difference in TEC with electrolyte (samarium doped ceria, SDC), and oxygen reduction reaction (ORR) kinetics were slowed as a trade-off [4]. The ORR consists of five steps: adsorption of oxygen molecules, dissociation of oxygen molecules, formation of oxygen ions, lattice mixing of oxygen ions, and migration of oxygen ions to the electrode/electrolyte interface. The ORR kinetics of BSF can be improved by doping other elements such as Cu, Zn, and Sb into the B-site to enhance reactivity with oxygen and promote oxygen ion conduction [2,5].
In this study, we investigated the electrochemical properties and ORR kinetics of Ba0.5Sr0.5Fe1-xCuxO3-δ (BSFCux) with the changes in oxygen vacancies and in electronic structure by doping Cu. The BSFCux were synthesized by a solid-state reaction, and all BSFCux was crystallized as cubic perovskite with a Pm-3m space group. In the Fourier transform data of the Fe-K edge obtained through X-ray absorption spectroscopy, the Fe-O peak intensity was decreased, indicating the increase in oxygen vacancy concentration of BSFCux with Cu doping. For the electronic structure of BSFCux, the average oxidation state of Fe was decreased from 3.584 (BSF) to 3.218 (BSFCu0.3), and the valence band maximum was calculated from valence band spectra, yielding −0.128 eV (BSF), −0.159 eV (BSFCu0.1), −0.185 eV (BSFCu0.2), −0.232 eV (BSFCu0.3). The BSFCux|SDC|BSFCux symmetrical cells were examined by electrochemical impedance spectroscopy (EIS), and BSFCu0.2 showed the smallest polarization resistance of 0.037 Ω cm2 at 700 ℃. The distribution of relaxation times (DRT) analysis for BSFCux will be discussed with its oxygen vacancy and valence band.
Fleming-O2
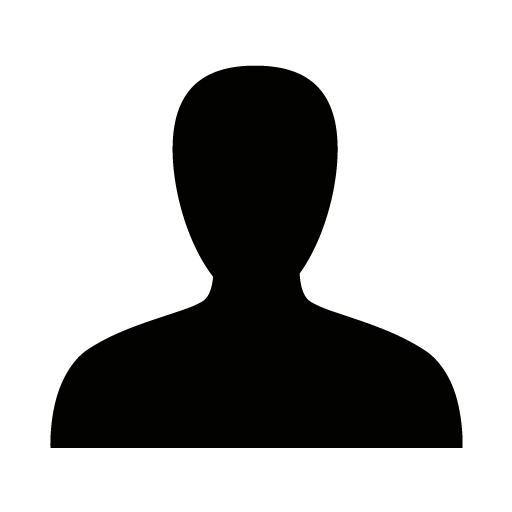
The experimental identification of the charge transition levels is of high interest, as it promotes the comprehension of the relationship between electronic structure and material properties. This serves as a foundation for quantitative defect models. The charge transition levels within the energy gap of transition metal oxides can be directly probed by means of X-ray photoelectron spectroscopy (XPS). They are directly connected to the oxidation state of the elements, dependent on the oxygen activity.
In this presentation, I will describe the recent work on identifying the charge transition level of Co2+/3+ in (La,Sr)CoO3-δ (LSCO) samples via X-ray photoelectron spectroscopy. All perovskite-type LSCO thin film samples have been deposited by RF sputtering. Post-deposition reducing surface treatments with water vapor and interface experiments to BaTiO3 (BTO) and Nb-doped SrTiO3 (STO) could reveal the Co2+/3+ charge transition levels, which are positioned at 0.01 eV above the valence band maximum. Therefore, the Fermi level in LSCO will be below the valence band maximum if Co is in the 3+ oxidation state. This is in good agreement with the high electronic conductivity of LSCO. Further oxidation of LSCO is expected to occur on the oxygen sites, as the presence of Co4+ states is not likely. The measured Co2+/3+ charge transition level of LSCO is consistent with the respective level in Co-doped BiFeO3 [1], assuming that the charge transition levels are aligned in perovskite-type oxides.
Fleming-O3
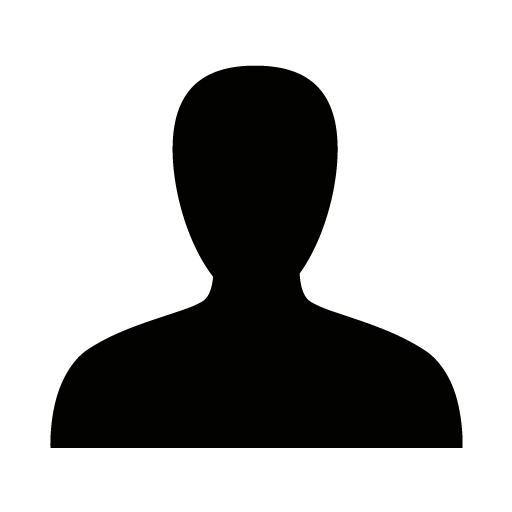
Cobalt-based perovskites, namely lanthanum strontium cobaltite (LSC) and cobaltite-ferrite (LSCF) are de-facto the state-of-the-art air electrode materials for SOC application. LSC and LSCF demonstrates good electronic conductivity, sufficient mobility of the oxygen ions and high electrocatalytic activity at reduced temperatures (LSCF down to 700C, and LSC potentially below 650C). However, a sufficient social-economical issue in usage of the Co-based materials is rising divergence between modest supply and growing demands. As result, Co is included in lists of the so-called critical raw material (CRM). These facts have boosted research on the effective low-Co and Co-free electrodes. One of the expected surrogates for cobalt is copper. As was already reported [1-3], partial or fully substitution of cobalt with copper might be a promising and economically relevant option.
In present work we studied challenges related to the preparation of air electrodes based on the selected materials from La1-xBaxCuO3-δ and ReBa0.5Sr0.5CoCuO5+δ (where: Re –lanthanides) solid solutions with perovskite structure. To prepare a properly sintered electrode that are mechanically stable, adheres to the electrolyte and at the same time to mitigate migration of alkali earth elements to the ZrO2-based electrolyte, it is crucial not only to conduct the sintering process at the optimal temperature profile, but also optimize buffer layer. The work involved studies of air electrode layer preparation on the semi-technical, 5 cm x 5 cm, fuel electrode-supported cells by changing the sintering temperature, atmosphere in furnace, implementation of the sintering aids a a modification of the buffer layer. Later one included co-sintering of the of lanthanum doped ceria (LDC) on a top of GDC. The SEM and SEM-EDS techniques were used to analyze the microstructure and products of the sold state diffusion of the Sr/Ba. Electrochemical characterization of the cells was based on electrochemical impedance measurements of the cells in SOFC and SOEC modes in gas-tight steel cell with gold air-side current collector and Crofer 22APU grill as fuel-side one in the temperature range of 650-750 °C. Results demonstrated, that mechanically stable air electrode from La1-xBaxCuO3-δ or ReBa0.5Sr0.5CoCuO5+δ cannot be fabricated on surface of the GDC as buffer layer without strong migration of the Sr and Ba toward 8YSZ electrolyte. Cells with dual LDC-GDC buffer demonstrated similar performance with LSC electrodes at 625-650 C. For the for the copper-based materials obtained performance might be considered as promising, however microstructural optimization of the layer is necessary to compete with state-of-the-art materials.
Fleming-O4
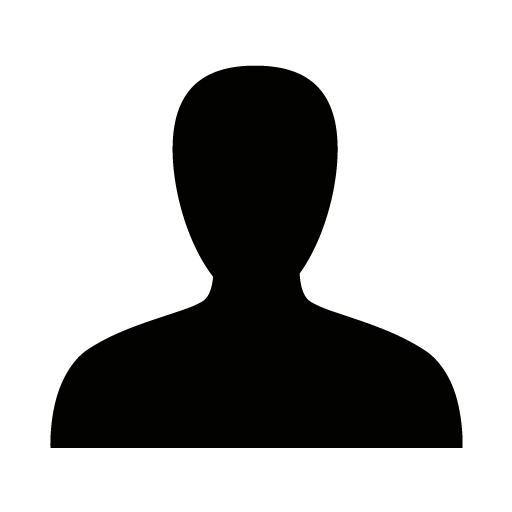
Investigating materials applied as oxygen electrodes in solid oxide cells (SOCs) is of great interest for energy sustainability. While SOCs are the most efficient technology for energy conversion, the materials utilized for the oxygen reaction are one of the main bottlenecks for their implementation in real systems, both in terms of performance and durability. The need to extend the material space explored to full material libraries is of critical interest in this field. Employing thin film combinatorial methods for the simultaneous growth of complete material libraries has been proven effective for obtaining self-sustained experimental datasets1,2. These approaches require the development of advanced material fabrication and characterization techniques.
In this contribution we have carried out a thorough study of a complete thin film (La,Sr)(Mn,Co,Fe)O3 (LSMCF) material library fabricated by combinatorial pulsed laser deposition. Combinatorial deposition allows the full characterization of the material library under the same conditions, reducing experimental uncertainty associated to sample variability. The library was characterized by XY-resolved X-ray fluorescence spectroscopy (XRF), X-ray diffraction (XRD), Raman spectroscopy and spectroscopic ellipsometry for mapping the compositional, crystallographic, structural and electronic properties The oxygen exchange kinetics at 400 °C was probed by means of isotopic exchange depth profiling coupled with secondary ion mass spectrometry (IEDP-SIMS). The electrochemical performance was studied by impedance spectroscopy (EIS), resulting in mapping the activation energies and area specific resistances for the oxygen reduction reaction in the 675-750 °C temperature range. The screening carried out represents an extended analysis covering the overall properties of the compositional family. Additionally, a series of selected material library compositions have been studied in terms of their stability against thermal degradation. Microstructural characterization, surface chemistry and cation depth profile analyses have been employed for analyzing the film degradation in terms of migration of strontium and formation of secondary species. The results indicate that the addition of Mn to LSCF-based electrodes plays a substantial role in the stabilization of strontium segregation phenomena throughout the material library.
Fleming-O5
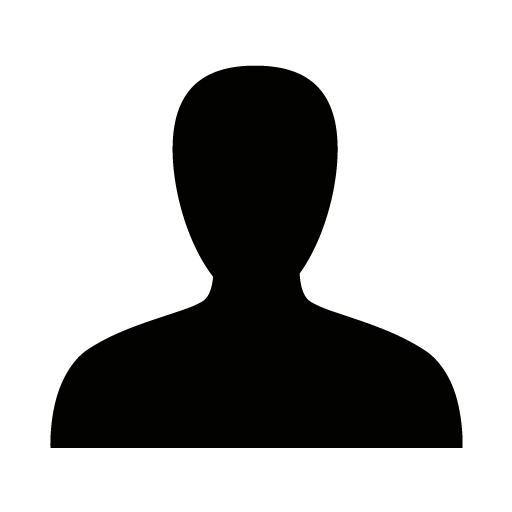
Paul holds B.Sc in Nanoscience and Nanotechnology from Univeristat Autònoma de Barcelona (2021) and a M.Sc in Quantum Science and Technology from Universitat de Barcelona (2022).
During his studies he received the ERASMUS-scholarship and had the opportunity to work on Organic Photovoltaic (OPV) at the CNRS-Strasbourg. During his master, he worked on Optomechanics in the Catalan institute of Nanoscience and Nanotechnology (ICN2, Barcelona).
He is currently carrying out a PhD at the Catalonia Institute for Energy Research (IREC, Barcelona) on functional and voltage-switchable thin film materials.
Recent predictions highlight that the exponential increase of computational operation could present a major share of energy consumption by 2050. To overcome this problem, new and energy efficient computing elements and logics must be developed. The possibility of changing the properties of materials by voltage-driven ion migration represents a promising approach to reach ultralow-power in storage and computing devices. An excellent example of the modulability of functional properties can be found in hole-doped perovskite ferrites, La1-xSrxFeO3-δ (LSF) thin films. Control on their properties is usually achieved either by A-side doping (x) or change of the oxygen vacancy concentration (δ) [1, 2]. In order for these materials to be implemented in next-generation devices, however, a detailed understanding and control of the underlying mechanisms controlling the stoichiometry-functionality relationship is of outmost importance.
In this work, we present the tuning of the oxygen vacancy concentration δ as a powerful tool for the careful control of electrical conductivity and related properties in the La1-xSrxFeO3-δ (LSF) family. This effect is achieved by voltage-driven (de)intercalation of oxygen ions in a robust, fast, and reversible way in highly oriented LSF films deposited on a solid electrolyte. Two exemplary materials in this family were chosen for a comparative study: SFO (SrFeO3-δ) and LSF50 (La0.5Sr0.5FeO3-δ). We show that potentially infinite intermediate states, characterized by different electronic conductivity, can be stabilized by changing the oxygen content in a continuous and controlled way (from a conductivity of 10-3Scm-1 to an increase of up to 5 orders of magnitude for both LSF50 and SFO measured at 50°C, and a measurable change as low as 2·10-3Scm-1). This holds true for both SFO – which presents a metal-to-insulator transition based on its topotactic transformation from a perovskite to a stabilized brownmillerite depending on δ – but also for LSF, i.e. within the single-phase domain. This represents a remarkable step forward with respect to the state of the art, where for example SFO is usually treated as a simple binary system, and make the SFO-LSF family a potential candidate e.g. for optical-based devices and analog memories. A smart experimental design allowed for the simultaneous characterization of structural, optical, electrical, and magnetic properties of the films, shedding light on the mechanisms that correlate such properties with the oxygen content and temperature. These results reveal clear trends that can serve as a guide for the implementation of future devices based on the LSF group materials.
Fleming-O6
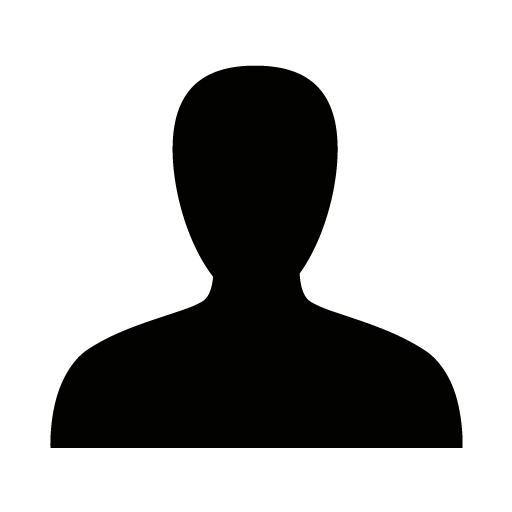
The efficiency of solid oxide fuel cells is significantly influenced by the rate of the oxygen reduction reaction (ORR) occurring at the cathode[1]. Addressing this challenge, alongside the development of entirely new materials, involves the chemical doping of pre-existing old material. In this context, doped BaCoO3-δ (BCO) perovskites have gained attention as versatile electrocatalysts at lower operating temperatures, surpassing the limitation of conventional material such as SrCoO3-δ based perovskites[2]. However, the role of dopants remains ambiguous and requires further investigation.
Here, we present a comprehensive study involving various dopants to understand cubic stabilization, focusing on the formation of mobile oxygen vacancies and oxygen adsorption on the cobaltite perovskite. The transition from a hexagonal to cubic structure not only promotes the formation of abundant mobile oxygen vacancies but also enhances the oxygen adsorption on the surface, which markedly facilitate the oxygen reduction reaction. Once stabilized into the defective cubic structure, the electrochemical activity was determined by the type of dopant. Interestingly, donor-doped BCO stands out as the most promising candidate among those considered particularly at the relatively high temperature, striking a delicate balance between a high concentration of oxygen vacancies and resulting phase instability. This differs significantly from conventional high-performance ORR electrocatalyst design strategies.
Westminster-O1
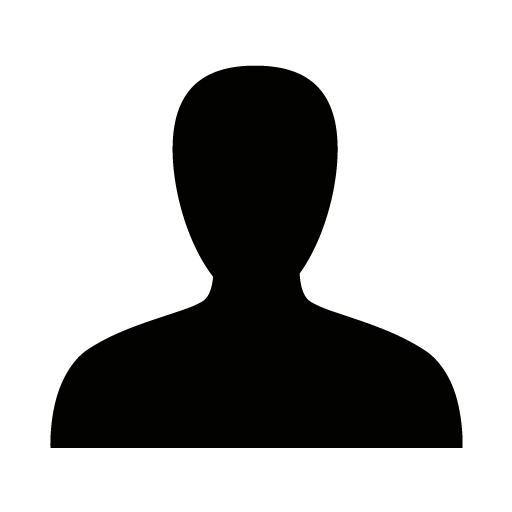
This study investigates the LaxNa1-3xNbO3 perovskite solid solution formed between nominally A-site
occupied NaNbO3 and highly A-site deficient La1/3NbO3 end members. Specifically, this work aims to
create A-site vacancies, introduced within the solid solution as charge compensating defects
consequent of the trivalent La3+ substitution for Na+ in the ABO3 perovskite structure, and to correlate
their influence on the dielectric properties observed.
Conventional dielectric spectroscopy (DS) analysis reveals a noticeable suppression
of the permittivity’s temperature dependence, alongside an associated antiferroelectric-relaxor type
transition, as the A-site vacancy (VNa’) concentration increases. This antiferroelectric-relaxor transition
can be classified as an order-disorder type transition at La concentrations of 7.5mol% and above, and is
reflected in significant changes to long range polarisation correlations. Structural
investigations involving Rietveld analysis of laboratory X-ray diffraction data reveal a series of phase
transitions across the solid solution, with notable diffuse scattering offering insight into the disruption
of long-range ordering associated with the NaNbO3 end member as La/VNa’ concentrations increase –
something that may be responsible for the observed flattening in the dielectric response with
temperature. La doping beyond 15mol% results in a quasi-linear type permittivity response, which is retained for the rest of the solid solution - up to and including the La1/3NbO3 end member.
Impedance Spectroscopy (IS) measurements suggest good dielectric
behaviour (electronically insulating behaviour) can be retained for low doping concentrations of La
(0<x<0.15), complementary to low dielectric loss data from DS measurements. Concentrations above
this threshold are proposed to induce ionic conduction with a maximum conductivity of ~6.3x10-5 Scm -1 at
300°C for a composition for La0.25Na0.25NbO3 . This ionic conductivity correlates well with the high
dielectric loss observed for these materials by DS measurements.
Westminster-O2
Mastering the behavior of mobile ionic defects in photo-active mixed ionic-electronic conductors exposed to light and/or voltage bias is one of the most exciting frontiers in solid state ionics. It represents both a conceptual challenge, as it requires combining knowledge on the defect chemical and optoelectronic properties of materials, as well as a promising perspective, as it may pave the way to novel devices in the field of energy and information technology.[1][2] Progress in this direction relies on the development of appropriate models that can provide an accessible, yet accurate, interpretation of experimental results in terms of material properties and device function. In practice, this means designing tools able to predict and analyze (i) the behavior (such as the electrical response) of devices based on mixed conducting materials, and (ii) trace such behavior back to the transport, storage and reaction of defects, described as function of the relevant thermodynamic parameters and bias condition. In this contribution, we address these aspects.
First, we propose an equivalent circuit model that can describe the electrical response of devices based on mixed conductors under bias. The circuit is a generalized version of previously reported models combining the established transmission line approach[3] with the concept of ionic-to-electronic current amplification.[4] We demonstrate the analytical accuracy of the model, by comparing it with drift-diffusion simulations of mixed conducting devices. We focus on hybrid halide perovskites as model systems. This material class presents excellent optoelectronic properties, and also shows significant ionic conduction even at room temperature.[5] Our analysis of calculated impedance spectra based on the proposed model allows the clarification of several anomalous experimental observations, including inductive behavior and multiple low frequency features reported for perovskite solar cells.[6]
Secondly, we focus on questions related with the quasi-equilibrium of photo-active mixed conductors under light, in terms of their defect behavior. By combining defect chemical models describing hybrid perovskites[7][8] with the relevant description of their optoelectronic properties, we are able to explore the effect of light on the steady-state defect concentrations. We present a systematic analysis of the key parameters in our kinetic model. Specifically, we consider the role of ionic disorder, electron-hole generation and recombination, redox and surface reactions in the system. The results highlight guidelines for the design of materials used in solar cells. More in general, they point towards the potential of controlling the defect chemistry of active materials (e.g. via control of component partial pressures) to optimize the performance of solar energy conversion devices.
These findings will aid the progress towards a complete description of the role that light plays in the already complex interplay between ionic and electronic defects in the solid state. The proposed models can be applied to the investigation of mixed conducting devices employed in applications ranging from energy conversion and storage to computing and sensing.
Westminster-O3
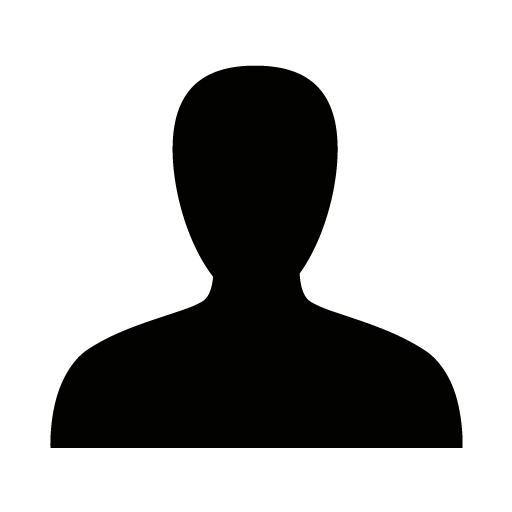
Space charges at the interfaces in optoelectronic devices significantly influence charge carrier dynamics, such as charge carrier transport and recombination, thereby affecting device efficiency.
CH3NH3PbI3 (MAPI) is a mixed-ionic electronic conductor, where iodide vacancies are the majority ionic mobile carriers, with minor contribution to ion conduction from Pb2+ and MA+ defects [1]. Due to its mixed conducting property, it is essential to consider both electronic and ionic interactions and their impact on space charge. However, while electronic equilibration (Fermi level alignment) is the established method to analyze equilibrium space charges in solar cell materials, including ionic interactions in the analysis of interfaces within MAPI based is yet largely overlooked.
First, we discuss the experimental investigation of ionically-generated space charges in MAPI when it is in contact with alumina. Zeta potential measurements are performed on alumina nanoparticles immersed in electrolyte containing salts relevant to MAPI, and they indicate a strong ion interaction between alumina and positive charged ions in MAPI precursor solution. These are expected to adsorb on their surface, result in the depletion of positive charge carriers and accumulation of negative charge carriers in MAPI in proximity of its interface with alumina nanoparticles. Using conductivity measurements in composite thin films as a function of the iodine partial pressure, we demonstrate that such interfacial ionic equilibration induces space charge potentials in the order of 700 – 800 mV at equilibrium, consistent with our previous report [2]. We then investigate the role of surface modification using chemisorbed organic molecular monolayers on alumina in altering the space charge situation within MAPI. Zeta potential results show that the ionic interaction can be modified through the surface modification of alumina, which we assign to a reduced ionic adsorption induced by steric effects or changes in the alumina surface chemistry. The results confirm the formation of reduced ionic space charge potentials in MAPI for the films with surface modified alumina, demonstrating that molecules adsorbed on the surface of contact phases are a promising tool to tune the space charge situation at the interfaces with MAPI.
Finally, extending this strategy to solar cell devices, we discuss the role of ionically-generated space charge regions in the active layer using the investigated alumina:MAPI composites. We demonstrate the detrimental role of having distributed p-n junction in the active layer for device performance, an effect that can be mitigated by surface modification. Finally, we address the impact of space charges and their modulation in MAPI infiltrated within mesoporous TiO2 layers, a commonly adopted electron transport layer in MAPI based solar cells. This study will help the design of perovskite devices with improved interfacial properties and the interpretation of experimental data accounting for the mixed conducting nature of halide perovskites.
Westminster-O4
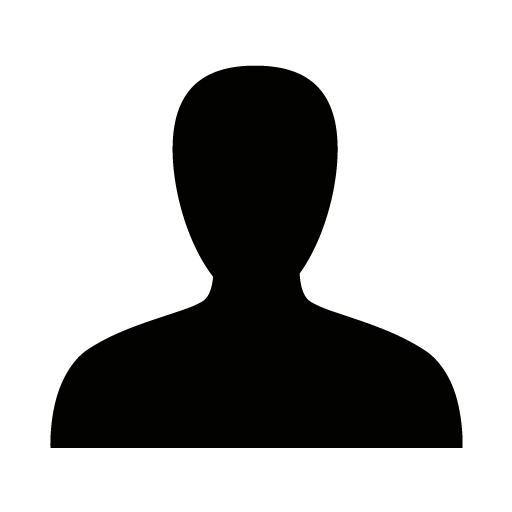
Using mixtures of iodide and bromide in halide perovskites has proved to be a powerful tool for tunable optoelectronic material design. However, such mixtures suffer from photo-induced phase segregation when exposed to light (photo de-mixing)[1]. While the process is reversible (two phases remix back in the dark to the pristine state, dark re-mixing),[2] it can potentially lead to unstable optoelectronic properties and device performance during operation, making its understanding essential to progress the field of halide perovskites. Since the observed light-induced phase separation involves significant ion transport, clarifying the ionic and electronic transport properties and therefore underlying defect chemical mechanisms involved in photo de-mixing is crucial.
Here, mixed iodide-bromide perovskites with different dimensionalities (2D, 3D) are investigated, in terms of phase and charge transport properties using a wide range of experimental techniques as well as theoretical calculations. We focus on 2D Dion-Jacobson mixed bromide-iodide perovskites (PDMA)Pb(Br0.5I0.5)4 (PDMA: 1,4-phenylenedimethanammonium spacer) as a model system to study photo de-mixing, due to its established reversibility.[2] First, we track the compositional evolution in the films during de-mixing and re-mixing by analyzing their time-dependent in-situ optical absorption properties. We also simultaneously monitor the conductivity changes during de-mixing and re-mixing, which allows for a local probe of the ionic and electronic charge carriers concentration and ion transport through the de-mixed phases. The dependence of the phase behavior and charge transport properties of mixed halide perovskites by varying dimensionality and surface conditions is further revealed using similar techniques. Furthermore, we take advantage of SEM and TEM to investigate the morphological changes and the nature of the iodide-rich and bromide-rich phases resulting from phase segregation. Lastly, we propose a model that considers possible opto-ionic effects, which can contribute to the driving force of de-mixing[3-4] and should therefore be considered in the overall energy balance of the process, together with the electronic effects discussed in the literature.[5] Our work sheds light on fundamental questions related to the phase behavior and the role of defects on the driving force of de-mixing. These findings will also aid compositional engineering related to halide mixtures, which will enable the optimization of optoelectronic devices as well as the development of other emerging systems exploiting photo de-mixing or in general photo-ionic effects.
Westminster-O5
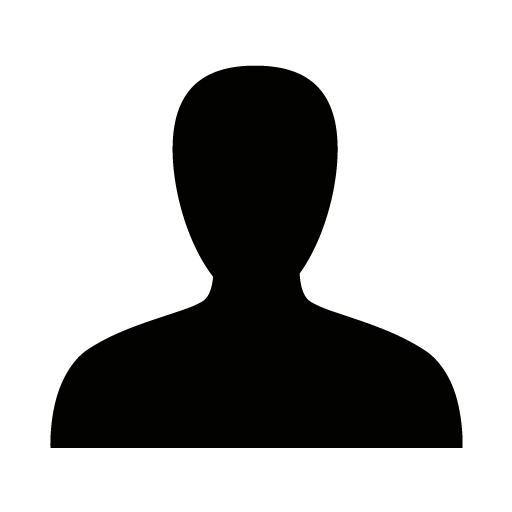
Perovskite lead–halides (PLHs) are approximately an order of magnitude softer than their oxide counterparts. While this property (among others) makes them promising candidates for applications in e.g. flexible (opto-)electronic devices, there is a strong correlation between mechanical strain and the device stability, which has been attributed to changes in the activation energies for ion transport in the PLH layer due to residual strain, and for anion vacancy transport in particular [1,2]. On the other hand, the interplay between strain and ion transport could be exploited for e.g. memristive applications [3]. While there exists computational evidence that activation energies for anion-vacancy hopping are lowered in the presence of positive (tensile) strain and increased in the presence of negative (compressive) strain [4,5,6], a detailed atomistic analysis of this interplay is currently lacking, and cation transport has not been considered at all.
Therefore, we carried out a DFT-based study on the effects of imposing biaxial strain on Br- and Cs-vacancy diffusion in a model perovskite halide, orthorhombic (Pnma) CsPbBr3. We calculate the activation energies for the hopping of vacancies between all pairs of nearest-neighbour lattice sites, and use the resulting values to parametrise a kinetic scheme, and thereby to calculate vacancy diffusivity tensors.
Our results indicate that the relationship between strain and vacancy diffusion is significantly more complex than previously thought – activation energies for vacancy hopping may increase or decrease for both negative (compressive) or positive (tensile) imposed biaxial strain, depending both on the plane in which strain is imposed and the particular pair of sites between which the vacancy hops, and the relationships are non-linear in general and often non-monotonic. Furthermore, we find that the influence of imposed biaxial strain on the diffusivity is significantly greater for Cs vacancies than for Br vacancies, and in particular, that values of Cs-vacancy diffusivity approach those of Br-vacancy diffusivity under certain conditions. This suggests that phenomena attributed solely to the transport of anion vacancies in PLHs might owe a significant contribution to cation-vacancy transport, particularly if a strong strain dependence is observed, such as in the stability of PLH based devices.
St.James-I1
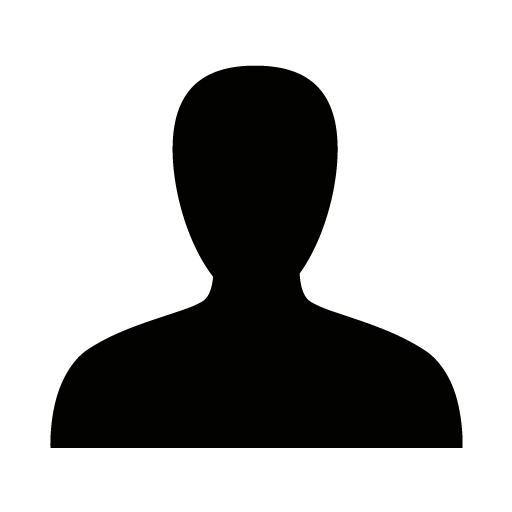
Proton ceramic electrochemical cells (PCECs) are emerging as a promising energy conversion technology for clean production of power (fuel cells), hydrogen (electrolyser) and chemicals (i.e., NH3 synthesis) – particularly due to their potential for intermediate temperature of operation (400-500°C). One of the main obstacles towards realizing intermediate-temperature operation is the kinetics of the O2/H2O-electrode (positrode). An emergence of novel positrodes based on mixed proton electron conducting oxides have resulted in excellent performance with electrode resistances below 0.5 Ωcm2 at temperatures above 500°C. However, the fundamental electrochemistry of positrode reaction mechanisms and the rate limiting surface reactions are not well understood, particularly the role of bulk and surface protonic species in the elementary reaction steps. Most electrode studies are performed under conditions in which the electrolyte material (typically BZCY-based) is a mixed conductor with comparable transport numbers for electron holes and protons (and to a certain extent oxide ions). Accordingly, the apparent electrode resistances due to accurately reflect the proton-mediated electrochemical processes which will occur in pure proton ceramic electrochemical cell.
In this work, we present a detailed study on interdigitated model electrodes (IDEs) prepared by PLD and lithography to create geometries with controlled electrochemically active area and triple-phase boundary length, with BGLC37 as the model electrode material. The electrode processes area measured at intermediate temperatures (200-500°C), low pO2 (< 0.05 bar) and high pH2O (>0.1 bar) to ensure the reactions are proton-dominated. A combination of standard EIS with voltammetry and Butler-Volmer analysis is employed to discriminate the true DC-polarization resistance and the separate time-resolved contributions occurring at or along the electrode surface. The surface species present and active on the electrode surface during operation is investigated using in-situ and operando XPS and XAS analysis and corroborated by ab-initio DFT simulations. The composition and structure of the electrode surface and the electrode-electrolyte interface is established by (S)TEM-EELS characterization of cross-sectional pieces of the samples. Finally, the impact of surface protonic species and their diffusion (surface protonics) on positrodes for PCECs is discussed based on combined input from DFT modelling and experimental evidence.
St.James-O1
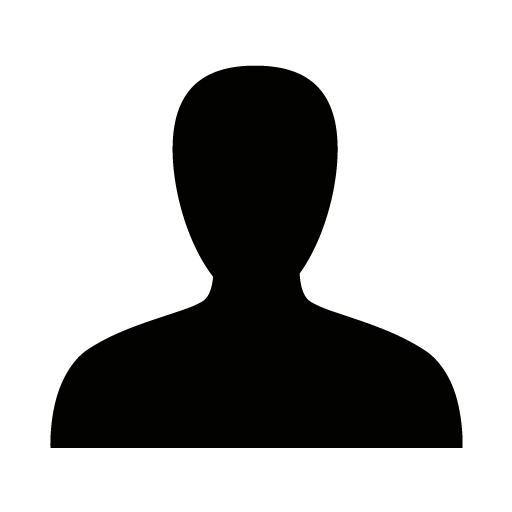
Energy storage systems are indispensable to power electric devices such as mobile phones, electric vehicles, and smart grids. Lithium-ion battery dominates the largest segment of the energy storage system market owing to its high energy density and long cycle life. However, flammable organic liquid electrolytes are used in lithium-ion batteries, which is an essential risk of fire and explosion accidents. Substituting water solvent for organic solvent directly approaches the safety issue. In particular, using proton as a charge carrier enables denser charge storage in electrode materials compared with other cations because proton possesses the smallest ionic radius. Therefore, aqueous proton battery is enthusiastically developed as an alternative to lithium-ion batteries.1
In general, acidic electrolyte shows intense hydrogen evolution reaction (HER) just below 0 V vs SHE because of its high activity of proton. To suppress HER in aqueous rechargeable batteries, molecular crowding electrolytes can be used, where mixed crowding agents (macromolecules or small hydrophilic molecules) reduces activities of H2O/H3O+ at electrode-electrolyte interfaces.2,3 Meanwhile, active materials should also possess high durability against physical and chemical change upon charge/discharge. Especially, large volume expansion/shrinkage usually raise intra-particle cracking on active materials, which exacerbates parasitic reactions at electrode-electrolyte interfaces.
In this presentation, we show that Mo3Nb2O14 exhibits reversible five-proton intercalation in an acidic molecular crowding electrolyte. The strain-free nature of Mo3Nb2O14 upon charge/discharge per the formula unit (ΔV/V < 0.5%) is confirmed by 1H solid-state nuclear magnetic resonance spectroscopy, X-ray absorption spectroscopy, X-ray diffraction, atomic-resolution scanning transmission electron microscopy, and density functional theory calculations. As a consequence, intra-particle cracking is suppressed on Mo3Nb2O14 particles, which minimizes HER to achieve high Coulombic efficiencies (> 99.7%). Finally, a full-cell that consists of the Mo3Nb2O14 anode, the prussian blue analogue vanadium hexacyanoferrate cathode 4, and the acidic molecular crowding electrolyte retains 98.5% of the initial specific capacity after 1000 cycles.
St.James-O2
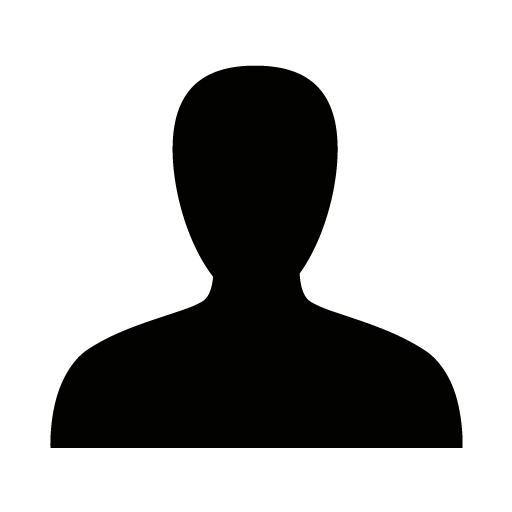
Solid oxide cells (SOCs), which replace oxygen ion conductors with proton conductors, are called protonic ceramic cells (PCCs) and are emerging as a game changer in the clean energy market due to the promising feasibility of low-temperature operation. PCC has similarities to SOC in cell structure and material selection. Therefore, it was expected that rapid progress would be achieved based on many previous studies. However, except for a few representative outcomes, PCC does not show a clear comparative advantage in performance compared to SOC. Despite the advantages of the electrolyte, the electrochemical activity of the electrodes, especially the air electrode, has a significant impact on the overall cell performance. Therefore, to overcome the aforementioned limitation, an in-depth understanding of the electrochemical reactions occurring at the electrode must be preceded, which can provide fundamental insights for designing highly active electrodes. Nevertheless, due to the complexity of the reactions occurring in the air electrode, few related studies have been reported.
In this work, we perform a case study using a geometrically well-controlled thin film-based model electrochemical cell fabricated by pulsed laser deposition (PLD). BaFeO3-based perovskite oxide was chosen as the air electrode material, and a current collector was embedded (Pt pattern patterned with photolithography) to selectively observe only the surface reaction at the thin film and gas interface. In particular, we present a reliable electrochemical model platform in which surface degradation of Ba-containing air electrode materials and electrolyte hole leakage in a single chamber setup are effectively suppressed. Using this, the active region and reaction pathway are observed through electrochemical characterization (electrochemical impedance spectroscopy) and real-time surface chemical analysis (ambient pressure X-ray photoelectron spectroscopy) under various gas partial pressures & overpotentials. Our study contributes to building mechanistic guidelines for designing highly active air electrodes for protonic ceramic cells.
St.James-O3
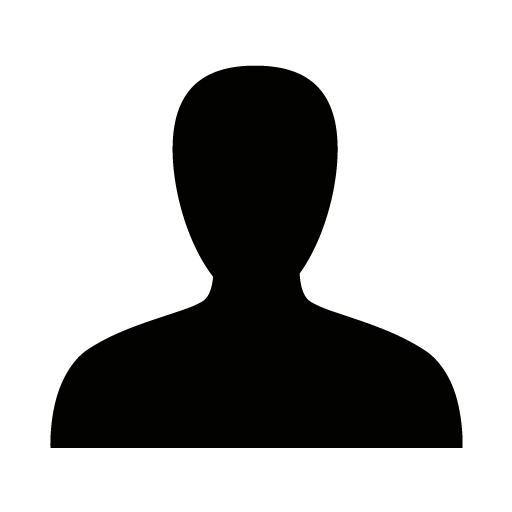
The main theme of this presentation is to cross-link several techniques including DFT calculations and neutron techniques to discuss the medium-low temperature (150℃ to 450℃) protonic conduction in barium-based perovskite system.
Protonic conducting fuel cells (PCFC), compared with conventional oxide-ion conductors, generally possess the advantage of lower operating temperatures, due to the smaller activation energy required for the protonic hopping.
BaZr0.1Ce0.7Y0.1Yb0.1O3-δ (BZCYYb), reported by Yang et al. [1] was regarded as the promising PCFC due to its high ionic (mixed protonic and oxide-ion) conductivity (102 Scm-1 at 600 ℃) under humid condition. However, this measured conductivity was not solely the protonic part, and other components such as electronic conduction (from the change of oxidation state in cerium) also contribute. Moreover, there are few studies focusing on the medium-low-temperature (<500℃) protonic conduction, at which the PCFC should possess more advantages.
In order to evaluate the medium-low temperature protonic conduction of BZCYYb, four topics are included in this presentation: The effect of hydrogen incorporation (via hydration annealing) on the crystal structure by X-ray Diffraction (XRD) and Neutron Diffraction (ND); The localised proton motion probed by Density Functional Theory (DFT) calculation and Quasi-Elastic Neutron Scattering (QENS) techniques [2]; The long-range protonic diffusion measured by EIS and Isotopic-exchange Secondary-Ion Mass Spectrometry (SIMS) methods; The proton/hydration incorporation amounts measured by Thermogravimetric Analysis (TGA) and Neutron Prompt-gamma ray Activation Analysis [3] (PGAA) techniques.
As suggested in the Grotthuss mechanism[4], proton incorporation is accompanied with the hydrated condition. In first section (hydration incorporation effect), the change of crystal structure after hydration annealing will be discussed. Though many existing literature discovered the phase transition of BZCYYb with increasing temperature[5], the effect of hydration is not available. The high-temperature XRD complemented with ND technique are applied to study the relationship between hydration and phase transition.
BZCYYb achieves higher conductivity than its undoped primitive BaCeO3 with small percent of b-site doping, but the localised effect brought by this small degree of doping is not well discussed. With better understanding of the structure change with humid annealing, in second section (localised proton motion), the localised hopping information such as hopping distance, preferred route and activation energy will be suggested by complementing the QENS data with ab-initio molecular dynamics (AIMD) and Nudged Elastic Band (NEB) calculation.
Generally, the protonic conductivity will be measured by EIS under dry and humid condition, but the obtained conductivity can be affected by other terms such as electronic and oxide-ion conduction. In third section (long-range protonic diffusion), by applying the Nernst Einstein equation [6] the tracer coefficient of proton isotope measured by SIMS could suggest its corresponding protonic conductivity .
To connect the diffusion coefficient with conductivity by Nernst Einstein equation, the concentration of incorporated protons is needed, but the exact number of protons in BZCYYb system is missing. Though normally proton/hydration incorporation is measured by TGA in an indirect way [5], the measured mass change can be affected by other factors such as change in oxygen stoichiometry. In fourth part (amount of incorporated protons), the PGAA neutron technique is applied to measure the proton amount directly, hence to obtain the amount of hydrogen under different annealing conditions.
In summary, the unique points of this research is applying "proton-sensitive" techniques (which could locate directly the proton dynamics and incorporation amount) and cross-linking them to evaluate the medium-low temperature protonic conductivity of BZCYYb system.
St.James-O4
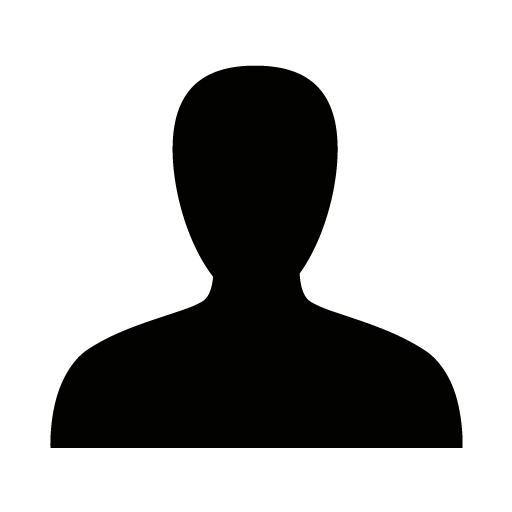
The next generation of proton exchange membrane fuel cells (PEMFCs) require a substantial reduction or elimination of Pt-based electrocatalyst from the cathode,[1], [2] where O2 reduction takes place. The most promising alternative to Pt is atomic Fe embedded in N-doped C (Fe-N-C). Successful incorporation of Fe-N-C in PEMFCs relies on a thorough understanding of the catalyst layer properties, both ex situ and in situ, with tailored electrode interface engineering.[3] Here, it is demonstrated that a previously developed high pore volume Fe-N-C [4] requires a sufficiently high ionomer to catalyst mass ratio (I/C, 2.8≤I/C≤4.2) for optimum PEMFC performance under H2/O2. Advanced in situ electrochemical techniques (distribution of relaxation times[5] and Fourier transform alternating current voltammetry[6]) were used to deconvolute for the first time the trade-off between proton and electron resistance and in situ FeNx active site density with increasing ionomer loading. These findings highlight the significant impact of tuning the I/C ratio based on the catalyst layer properties and feature the power of emerging in situ electrochemical tools for optimising performance in PEMFCs and other electrochemical devices.
Moore-I1
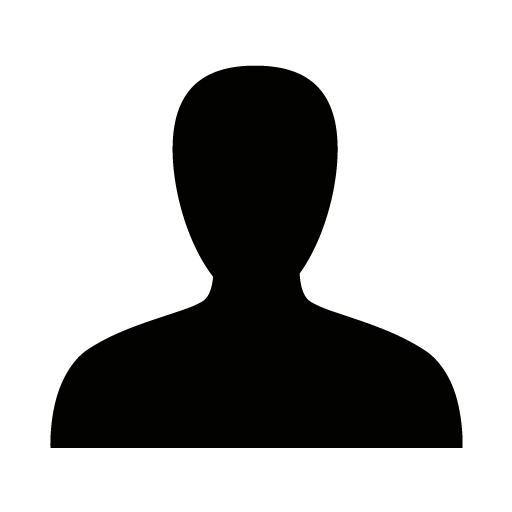
Solid oxide cells often employ a diffusion barrier layer, such as rare-earth doped ceria, to mitigate the chemical reaction occurring between common air-electrode materials such as lanthanum strontium cobalt ferrite (LSCF) and state-of-the-art yttria-stabilized zirconia (YSZ) electrolytes. In conventional cell fabrication methods, screen-printed gadolinia-doped ceria (GDC) is often co-sintered with YSZ at high temperatures typically exceeding 1300 °C, which inevitably results in the formation of an interdiffusion layer (IDL) at the GDC/YSZ heterointerfaces. This IDL has been identified as a ceria-zirconia solid solution which has significantly lower conductivity compared to either GDC or YSZ [1]. However, whilst it is commonly acknowledged that such interdiffusion may affect the overall cell properties, there is still a lack of understanding of its actual influence on oxide ion transport and cell degradation behavior.
In this study, we probed the effect of the IDL on oxide ion transport across GDC/YSZ heterointerfaces under fuel cell operation using the 18O oxygen isotope exchange method in conjunction with high-resolution secondary ion mass spectrometry (SIMS) imaging [2]. Two types of cells were examined: one utilizing porous screen-printed GDC interlayers (with IDL), and another utilizing dense GDC interlayers prepared using pulsed laser deposition (PLD; without IDL). A dramatic drop in the 18O tracer concentration was observed coinciding with the IDL location, thereby confirming that the IDL formed at screen-printed GDC/YSZ heterointerfaces inadvertently acts as a major barrier to oxide ion transport. The disruption of ionic flow is attributed to a possible effect of distribution and mobility of oxygen vacancies as induced by the mutual diffusion of cations across the GDC/YSZ heterointerfaces. On the other hand, using dense GDC interlayers prepared via physical vapor processes such as PLD avoids IDL formation, resulting in a remarkable improvement of ionic flow across the GDC/YSZ heterointerfaces. Typical ohmic resistance values for cells with IDL are also found to be higher than those without by approximately a factor of five, indicating that the cell’s increased ohmic resistance can be accounted for by the nature of the GDC interlayer and its associated interfacial properties. Accordingly, the effective mitigation of the IDL formation through an appropriate selection of cell fabrication parameters, specifically those employed for GDC interlayers, is considered as a good strategy towards further improving the performance of solid oxide cells.
Moore-O1
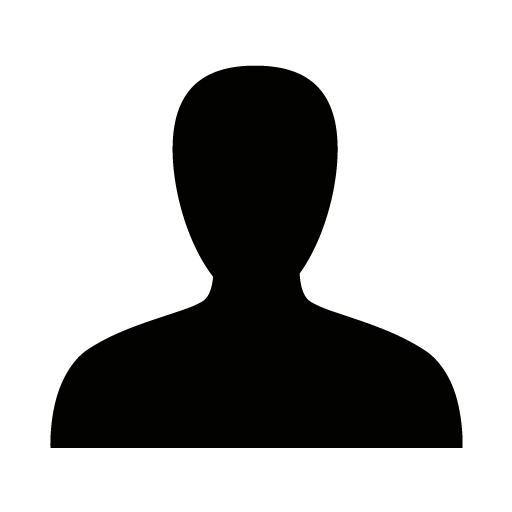
Interfaces are key to the properties of ionic materials. In particular, space charges at grain boundaries dictate grain boundary ionic resistance. In this talk, we use multislice electron ptychography to characterize atomic structure and space charge potential at grain boundaries in ionic conductors simultaneously. Existing methods for measuring space charge potentials at grain boundaries have significant limitations. For example, quantifying the potential present in space charge regions is challenging because these features are small—on the order of nanometers. Bulk measurement techniques like electrochemical impedance spectroscopy (EIS) fail to account for the diversity of grain boundaries and grain boundary properties present in bulk specimens. Existing transmission electron microscopy (TEM) and atom-probe tomography (APT) techniques are well-suited to chemical analysis of grain boundaries but provide only an indirect or semi-quantitative estimation of space charge potential. In this talk, we use multislice electron ptychography to characterize space charges at grain boundaries in Gd-doped CeO2. Multislice ptychography provides a 3D, quantitative measurement of potential, and is sensitive both to the high spatial frequency, strongly scattering atomic cores as well as the diffuse, weaker space charge potential—unlike other electron microscopy techniques. This allows the simultaneous reconstruction of atomic-resolution structural features and local space charge potential. Applied to ionic conductors, this allows observation of the coupling between grain boundary structure and electrochemical properties and, in turn, provides a path towards the design of materials with targeted interfacial properties.
Moore-O2
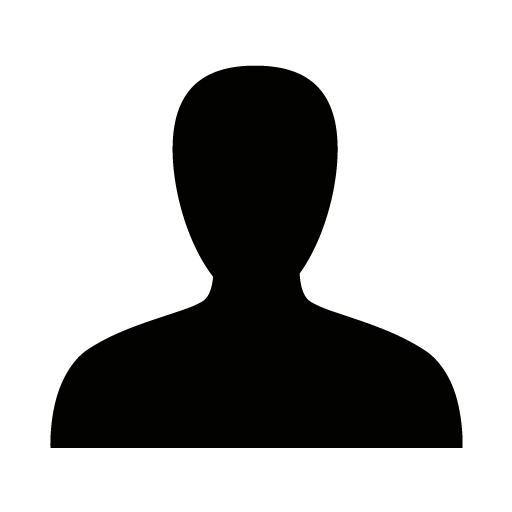
Solid oxide cells are promising devices for decarbonizing the energy economy, as they offer efficient energy storage and generate green alternatives to fossil fuels. In such electrochemical cells, the interfaces between various components are vital for the charge transfer kinetics and therefore for the overall performance of the cell. Although there are well-established models describing interfacial space charge regions for either electronic or ionic species, models describing space charge regions for both ionic and electronic charge carriers are far less developed. The latter type of space charge is, for example, present in high temperature solid oxide solar cells based on the interfaces between SrTiO3 (STO) and other mixed ionic and electronic conductors (MIECs) [1]. Experiments yield photovoltages up to > 1 V, but the interplay of ionic and electronic defects in determining the corresponding space charge potential is not truly understood.
This work focuses on the investigation of space charge regions between highly electronic conducting MIECs and STO. YBa2Cu3O7-δ (YBCO), La0.6Sr0.4FeO3-δ (LSF), La0.6Sr0.4CoO3-δ (LSC), La0.65Sr0.35MnO3-δ (LSM) and La0.9Sr0.1CrO3-δ (LSCr) thin films were grown by pulsed laser deposition on (nominally) undoped STO single crystals. The resulting interfacial space charge zones are investigated by several approaches: First and most prominently, the resistances of space charge regions at the MIEC│MIEC interfaces were determined in a broad range of oxygen partial pressures at 500°C by means of electrochemical impedance spectroscopy. According to these measurements, investigated materials are distinguishable into MIECs leading to very pronounced space charges (LSM, LSCr), MIECs with moderate space charges (LSF, LSC), and oxides that do not lead to any measurable space charge resistance (YBCO). Interestingly, experiments of nanometer thin interlayers of LSM revealed that already a layer thickness of a single unit cell leads to the formation of a space charge with properties very similar to much thicker films. In a second approach, 18O tracer diffusion across the corresponding interfaces revealed information on the depletion of ionic defects (oxygen vacancies). Third, work function measurements by means of X-ray photoelectron spectroscopy were performed for LSCr, LSM and LSF layers on STO, which strongly support the space charge data obtained from impedance measurements. The same is true for the fourth approach, the analysis of space charges by means of photovoltages measured upon UV illumination of these MIEC│STO hetero-interfaces [1]. Finally, a model is developed, which correlates the ionic and electronic driving forces determining the space charge potentials. This model predicts a relation between space charge potentials and reducibilities of the MIECs, i.e. the transition points in Brouwer diagrams. Comparison with literature data on defect energies indeed confirms the predicted trends.
Moore-O3
Triggering topotactic phase transitions by adjusting oxygen stoichiometry while maintaining the cation framework is recognized as an effective method for tuning functional oxide properties. This phase engineering can significantly alter the ligand field around metal cations and modify the electronic structure of oxides, potentially influencing (electro-)catalytic properties. Phase engineering is particularly relevant for tuning oxygen incorporation/evolution reactions (OIR/OER), crucial for energy conversion devices, especially solid oxide fuel/electrolysis cells (SOFC/SOECs). However, detailed case studies of mechanistic understanding of phase-dependent electro-catalytic activity, especially for oxygen exchange reactions at elevated temperature, have been rare. To tackle this challenge, in this presentation, we will show that crossing phase boundaries can lead to entirely different mechanisms of oxygen incorporation/evolution reactions, using a simple yet generalizable binary oxide, PrOx, as a model system.[1] We identify phase boundaries in PrOx, the threshold oxygen chemical potential triggering the phase transition, by measuring the electrochemical quantity of chemical capacitance. We found that the chemical capacitance reaches a maximum at the phase boundary. We also investigated the kinetics of oxygen exchange reactions, including incorporation and evolution, depending on the direction of applied biases. Surprisingly, crossing the phase boundary causes a drastic change in the reaction order concerning the pressure of the oxygen gas phase. The oxygen exchange kinetics show a strong dependence on the oxygen partial pressure for the oxygen-poor phase of PrOx, while for the oxygen-rich phase, the exchange kinetics are almost insensitive to the amount of available oxygen molecules. Therefore, we conclude that our findings pave the way towards a deeper understanding of phase-specific kinetics and reaction mechanisms for a variety of electrochemical interfaces.
Moore-O4
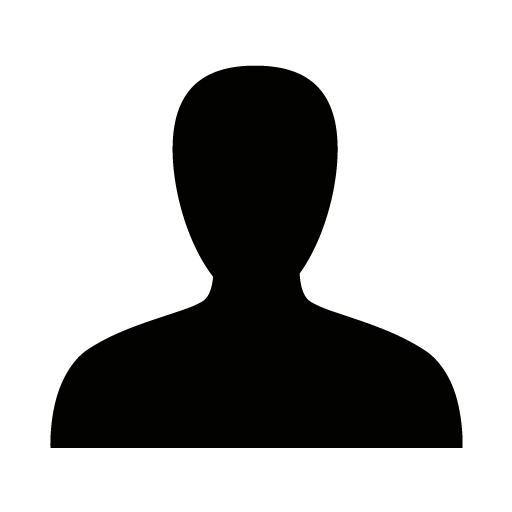
In the development of nano-scaled oxygen electrodes of the high temperature solid oxide cells (SOCs), the interface formed between the nanoelectrode particles and the electrolyte or electrolyte scaffolds is most critical for the high activity and high durability of SOCs. In this work, a new synthesis technique for the fabrication of nano-structured electrodes via the in situ electrochemical polarisation treatment is reported. To demonstrate the feasibility of the technique, lanthanum strontium cobalt ferrite (LSCF) nitrate precursor solution is infiltrated into gadolinia-doped ceria (GDC) scaffold pre-sintered on yttria-stabilized zirconia (YSZ) electrolyte, followed by in situ polarisation current treatment at operation temperatures in the range from 700 to 800℃. Results clearly show that both electrode ohmic resistance and polarisation resistance decrease with the increase of polarisation current treatment. Detailed microstructure analysis indicates the formation of convex-shaped interface between LSCF nanoparticles and GDC scaffold, very different from flat contact between LSCF and GDC observed after heated at 800oC with no polarization current treatment. Coherent lattice arrangement at the interface between LSCF and GDC are clearly revealed at the atomic level, contributing to the superior structure stability against long term cell operation. This is demonstrated by the excellent stability under both fuel cell and electrolysis operation conditions at 750oC. Moreover, good performance of in situ polarization induced nano-structured LSCF/GDC composite electrodes is demonstrated on single cells, achieving a high peak power density of 1.58 Wcm-2 at 750℃. This work highlights a novel and facile route to in-situ construct stable and high-performing nanostructured electrode for SOCs.
Abbey-I1
Louis Piper is Professor of Battery Innovation and heads the Battery Materials and Cells group at WMG. He is the PI for the FutureCat Phase II Faraday Institution consortium and also co-leads the Faraday Institution Degradation Phase II project with Dame Prof. Clare Gray (Cambridge). He has published almost 200 publications (h index = 61) and has various projects with industry partners and government agencies. Louis completed his PhD in surface Physics at Warwick University (2006) before moving to the USA. He was an Associate Research Professor for Boston University where we was also the beamline contact scientist at the NSLS (X1B beamline) at Brookhaven National Laboratory (2006-2010) He was then faculty at State University of New York, Binghamton (Assistant Professor to Full Professor, 2010-2020) wherhe was Director the MASE program and worked alongside Nobel Laureate Prof. M. Stanley Whittingham on several projects (most notably DOE EFRC NECCES). He returned to Warwick in 2020 and is focused on the circular economy of batteries from materials synthesis, cell scale up, advanced/operando characterization and recycling.
The manufacture of state-of-art Li-ion batteries require the consideration of $/KWh at cell and pack level at end of life (EOL) i.e. 80% capacity retention after 1000s of cycles. As a result, we need to simultaneously increase cell performance and longevity. Extending battery lifetimes in state-of-the-art batteries requires an academic understanding of degradation processes within industry-like manufactured cells. Ideally, we would like to directly observe the intercalation reactions directly within the real cells as a function of cycling to trace the origins of degradation. Unfortunately, most operando studies of these batteries employ either ½-coin cell configurations and/are compromised cells to cater the geometry of the X-ray experiments.
Here, I will summarize our recent developments to employ industry-like cells with in-house x-ray diffraction/absorption and electron microscopy to track the degradation in Ni-rich NMC (NMC811) // graphite single-layer pouch cells after prolonged cycling.[1] This new capability enables us to examine the fundamental intercalation reactions occurring in real cells under drive cycles for electric vehicle applications. As a result, it provides new insight into the origin of oxygen loss induced degradation. In state-of-art Ni-rich cell chemistries.[1,-4]
Abbey-O1
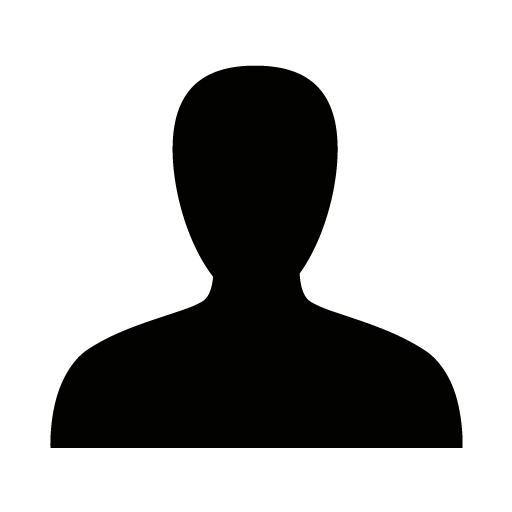
'Anode-free' Li metal batteries constitute a promising candidate for next-generation electrochemical energy storage devices. However, currently technologies suffer from rapid capacity loss due to uneven Li deposition and dissolution, resulting in continous electrolyte decomposition, loss of chunks of electrically isolated Li as 'dead Li' and also creating significant safety risks through the formation of short circuits.[1] A further issue is self-discharge after Li is plated onto anodic current collectors during charging. This occurs through two routes - reaction of Li metal with the electrolyte, and electrochemical galvanic corrosion where electroylte reduction happens via current collector, and Li is converted to Li+ - both pathways result in significant reduction in capacity if charged 'anode-free' batteries are left to rest. Prior work has established this phenomina as a result of differences in the SEI formation kinetics on Cu and plated Li metal,[1] and shown that the rate of corrosion may be altered through application of stable interphase coatings.[2] The current work utilises an approach combining Electrochemical Impedance Spectroscopy (EIS) with operando 7Li NMR to establih differences in corrosion behaviour and interphase evolution with different Li plating amounts. Assignment of features in the EIS Distribution of Relaxation Times profiles are made to cathodic interphase processes, and separate features are observed for Cu and Li interphase charge transfer. Interphasial capacitance values are extracted from EIS data through are recently developed general phase element analysis, providing information about charge transfer kinetics at Cu and Li interphases. SEM imaging is utilised to correlate observed differences in Li metal signal intensity in operando 7Li NMR with changes in plated Li morphology and the interphasial evolution tracked via EIS analysis. The developed workflow allows deconvolution of interphase evolution of Cu and Li using a simple experimental set up. This allows a deeper understanding of the exact mecahnisms for Li metal corrosion under different plated Li loadings, with higher loadings almost entirely initially suprresing Cu interphase evolution. The same methods are then applied to study novel developed inorganic-organic polymer coated 'anode-free' batteries - incooperating multi-strength polymeric cross-linking approaches to produce extreme elastomeric properties - showing improved corrosion resistance and interphase stability. This work highlights the potential application of polymer coatings to enable high performance 'anode-free' batteries and their ability to reduce self-discharge through Li corrosion.
Abbey-O2
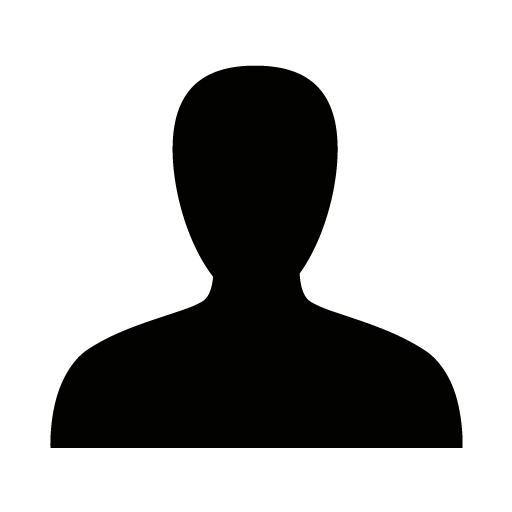
Anode-free solid-state batteries, in which the lithium anode is formed in situ during the first charge, could deliver a step-change in cell energy densities. However, at charge rates on the order of 1 mA cm2, plating of Li at the current collector/solid electrolyte interface is highly inhomogeneous, and dendrites (filaments of Li metal) are observed to penetrate through the solid electrolyte resulting in cell failure. Recent work has suggested that introduction of a silver-carbon composite interlayer between the solid electrolyte and current collector can prevent dendrite ingress into the solid electrolyte whilst promoting more homogeneous deposition of Li metal.[1]
In this work, we investigate silver-carbon composite interlayers, revealing the structural changes within the interlayer during charge and discharge, and the rate dependence of these changes. We go on to investigate the homogeneity of Li-Ag alloy and Li metal formation at the current collector with and without silver nanoparticles present in the interlayer, and how this affects the processes of charge and discharge. We demonstrate dendrite-free charge at 2 mA cm2 and reveal the origin of the failure of the interlayer at higher rates.[2]
Abbey-O3
Dr. Jongwoo Lim currently serves as an Associate Professor of Chemistry at Seoul National University. His academic journey began at POSTECH, where he obtained his undergraduate degree in Chemistry in 2008. He later pursued his Ph.D. in Chemistry from the University of California, Berkeley, graduating in 2013 under the guidance of Professor Peidong Yang. Post-Ph.D., Dr. Lim engaged in postdoctoral research with Professor William Chueh at Stanford University, before joining the Department of Chemistry at Seoul National University in 2017 as an Assistant Professor.
In all-solid-state batteries (ASSBs), (electro)chemo-mechanical aspects such as uneven interfaces, cathode-electrolyte interphase formation, delamination, fracture, defects, etc., are the major factors in capacity fade but remain largely unknown.
Nonhomogeneous transfer of lithium ions can cause significant variations in strain and stress within battery electrodes, leading to degradation in battery performance. In all-solid-state batteries (ASSBs), the lithium pathway and the associated strain/stress field become more intricate due to the (electro)chemo-mechanical reaction at the electrode-electrolyte interfaces. The dynamic volume change in active particles are heavily influencing by strength of the solid electrolyte and interfacial conformality. This can continually alter the lithium pathway and the internal stress field, leading to recurrent redefinitions of (electro)chemo-mechanical environment.
Here, we have developed an operando coherent X-ray imaging platform and associated analysis methodologies. This technology can track the nanoscale transport of lithium and the strain evolution of individual electrode particles in ASSBs. With this platform, we gained a comprehensive electrochemical and mechanical understanding of the cycling properties of single electrode particles in ASSBs.
Abbey-O4
Solid state lithium metal batteries are promising as the next-generation energy storage system due to their high energy density, thermal stability, and volumetric miniaturization.[1] However, the use of Li metal anode is detrimental in terms of dendrite formation and Li deposition in the solid electrolyte (SE) layer that causes short-circuit, thereby limits the battery cyclability. Besides, the mechanochemical instability of the Li-SE interface, and the low earth abundance of Li establish the need for alternative high-capacity anode development. In this regard, silicon is a potential alternative due to its high theoretical capacity of 4200 mAh/g and low lithiation potential. The realization of electrochemically stable and high-performance Si based anode is limited by low electronic and ionic conductivity of Si. [2, 3] Despite appreciable advances in achieving stable Si based anode, the influence of the transport properties on the rate capability and long-term stability remain unclear.
In this study, we investigated the role of effective ionic and electronic conductivity in modulating the electrochemical performance of Si based anodes. First, we have developed Si-based composites with SE (Argyrodite) and carbon additive, which significantly improved the ionic and electronic conductivity of the electrode composite, respectively. By optimizing the ion/electron conducting phase ratio in the composite and relating corresponding cell performance with effective transport coefficients we have estimated the limiting conductivity values. The particle size of Si is also found to significantly influence the effective transport properties, which in turn modulate the rate performance as well as the long-term stability.[4] This study provides a comprehensive understanding on the role of charge carrier transport in achieving high-performance silicon based anode for solid-state batteries.
Abbey-O5
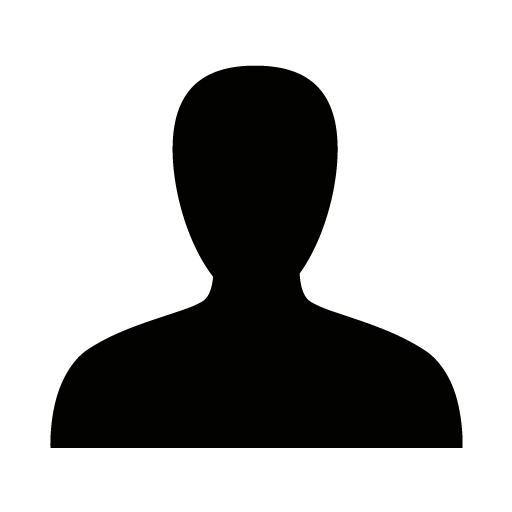
The overwhelming majority of layered oxide cathode materials are based on the redox activity of expensive and scarce transition metals like Co and Ni. While the Fe-redox based material LiFePO4 has become increasingly attractive due to its low cost, its low gravimetric energy density and relatively low operating voltage limit its use cases. A high-voltage Fe-based layered oxide cathode would provide high energy density utilizing the redox-activity of the most abundant transition metal in Earth’s crust, but such a material that provides stable and reversible electrochemical performance remains elusive. Indeed, high-voltage Fe-redox in oxides is plagued by cation disorder and oxygen dimerization, which leads to capacity fading and large voltage hysteresis (> 1 V).[1]
In contrast, the layered oxide cathode Li4FeSbO6 (equivalently, Li[Li1/3Fe1/3Sb1/3]O2, abbreviated LFSO) exhibits <0.2 V of hysteresis, and a discharge plateau at 4.2 V versus Li/Li+ with two-electron capacity.[2] Both the exceptional electrochemical reversibility of this material and its phase separating nature upon (de)intercalation raise questions about the origin of these properties that are unusual for layered Fe oxide cathodes. In this work, we will discuss our efforts to probe the electronic structure of LFSO with Fe L–edge x–ray absorption (XAS) and 57Fe Mössbauer spectroscopy, as well as O K–edge resonant inelastic x–ray scattering spectroscopy (RIXS). The combination of these element-specific and valence- state sensitive techniques, in conjunction with charge-transfer multiplet and density functional theory calculations, allows us to reveal the electronic structure changes at Fe and O as a function of state of charge for the first time. Correlating these electronic structure changes with synchrotron diffraction measurements, we reveal a unique redox compensation mechanism that readily rationalizes the exceptional electrochemical reversibility, unlocking a new direction for high-voltage, Fe-based redox systems.
Abbey-O6
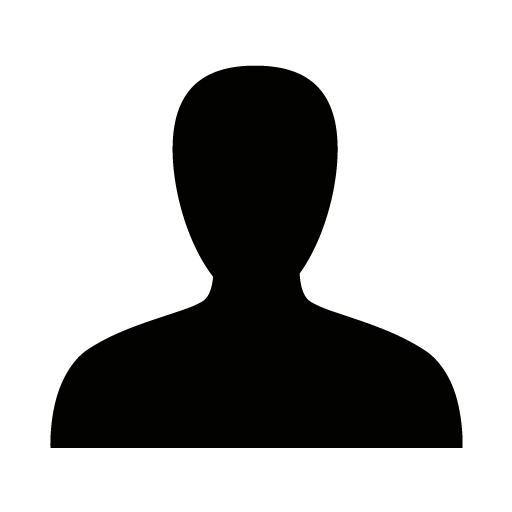
Electrolytes composed entirely of ions offer important safety and performance advantages over traditional molecular-solvent based systems, particularly for devices utilising reactive metals such as lithium or sodium. The benefits of ionic liquid (IL) based electrolytes are well recognised, while Organic Ionic Plastic Crystals (OIPCs) offer many of the same advantageous features and they are solid at room temperature.
An important approach to developing ionic electrolytes that can meet the complex challenges of next-generation electrochemical devices is increasing the range of known and well-characterised salt families. [1,2] It is recognised that the nature of the cations and anions used to make ionic liquid (IL) electrolytes can have a significant impact on the chemical, physical and electrochemical properties. The same is true for organic ionic plastic crystals (OIPCs); these salts are structurally analogous to ILs and but they are solid at room temperature and display dynamics that can allow their use as solid-state electrolytes. However, the structure-property relationships are arguably less well understood in OIPCs. Furthermore, the addition of different lithium or sodium salts to these materials, to enable their use in lithium or sodium batteries, introduces further complexities in terms of understanding and optimising the thermal, electrochemical and transport properties.
The field of ionic electrolytes can be further expanded by tethering the cation and anion together to form zwitterions. The development of zwitterions with molecular disorder allows their use as the main electrolyte matrix or as additives.[3]
Here we discuss our development of new plastic crystal-based materials for energy applications, in particular lithium metal batteries. We also discuss our recent research into novel zwitterion-based electrolytes. Insights into the relationships between the different ionic and zwitterionic structures and the physical, thermal and electrochemical properties of the resultant electrolytes are presented.
Gielgud-I1
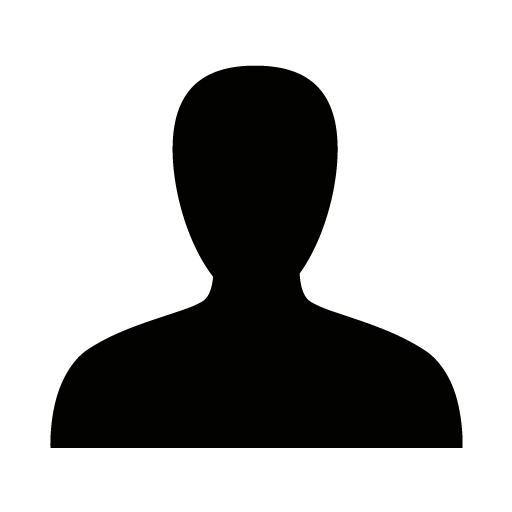
In this talk, a computational method for the training of digital twins is presented which allows for the generation of virtual microstructures of all-solid-state-battery (ASSB) cathodes by stochastic simulation. To ensure that digital twins are realistic, they are calibrated by means of 3D microscopy image data, which stem from focused ion beam-scanning electron microscopy (FIB-SEM). The ASSB cathode consists of single crystal LiNi0.83Co0.11Mn0.06O2 active material and thiophosphate-based glassy Li3PS4-0.5LiI solid electrolyte, treated with various milling media which results in different solid electrolyte particle size distributions and different electrochemical performance. After calibration of the digital twins to FIB-SEM data, virtually generated microstructures resemble real ones [1-3].
Additionally, by means of systematic variation of a digital twin’s parameters, we can generate a large database of different structural scenarios (like various volume fractions of solid electrolyte, active material or pores). These virtual microstructures can then be used as realistic geometry input for numerical simulations of electrochemical properties [4,5]. In this way, combining the results of stochastic and numerical simulations, we are able to investigate the influence of morphological descriptors (like active material size and shape, particle porosity caused by cracks, electrode porosity, average shortest path lengths) of the microstructure onto functional properties like electrochemical performance. In other words, a digital twin enables the investigation of quantitative structure-property relationships [1] based on computer experiments, which in turn can reduce costs in both time and resources.
Recently, purely data-driven digital twins have emerged, namely, generative adversarial networks (GANs), which are flexible computational tools for the generation of rather complex microstructures [6]. However, after training (calibration) it is difficult to systematically vary the GAN’s parameters for the generation of microstructures with different morphologies, which is an important step for the creation of a database that is sufficiently large for deriving structure-property relationships. An alternative to GANs are interpretable models from stochastic geometry [7] like excursion sets of Gaussian random fields (GRFs) which can be parameterized easily, thus, enabling a systematic variation of parameters for the investigation of various structural scenarios. But, such “simple” GRF-based models from stochastic geometry do not suffice as digital twins of the image data considered in this talk, since ASSB cathodes exhibit a rather complex microstructure.
Therefore, we combine both approaches, namely GANs and more “advanced” models from stochastic geometry, i.e., excursion sets of more general random fields. These models are (i) flexible enough to suffice as digital twins of ASSB cathode microstructures and (ii) parametric for enabling systematic variation of parameters in order to investigate various structural scenarios. As the calibration of the “advanced” models’ parameters to image data can be quite challenging using conventional methods of spatial stochastic modeling, we leverage the generative adversarial framework of GANs for the purpose of model calibration (i.e., the model tries to generate virtual microstructures that “fool” the so-called discriminator network). Thus, the “advanced model” can be used to generate (i) virtual, but realistic ASSB cathode microstructures as well as (ii) a broad spectrum of virtual materials with different morphologies—an essential prerequisite for deriving structure-property relationships.
Gielgud-O1
The development of safe, sustainable, and mass-deployable solid-state batteries requires fast-ion conducting solid electrolytes derived from earth-abundant elements. In the search for new solid electrolytes, a common approach is to alter the composition of already known phases by doping on anion or cation sites to modify the structure and conductivity. However, searching for new high-conductivity phases from structural modification only is less common. Motivated by this idea, we have implemented a computational materials discovery and screening procedure that combines first-principles structure prediction with machine-learning-derived force-field molecular dynamics. By applying this procedure to the Li–Si–S phase space, we find 21 phases within 30 meV/atom of the convex hull. Four of these phases are predicted to show high Li-ion conductivities, of which three structures are previously unknown and are targets for future synthesis. This combined computational structure prediction and screening methodology holds promise for use in the discovery of novel fast-ion conductors beyond the Li–Si–S chemical space.
Gielgud-O2
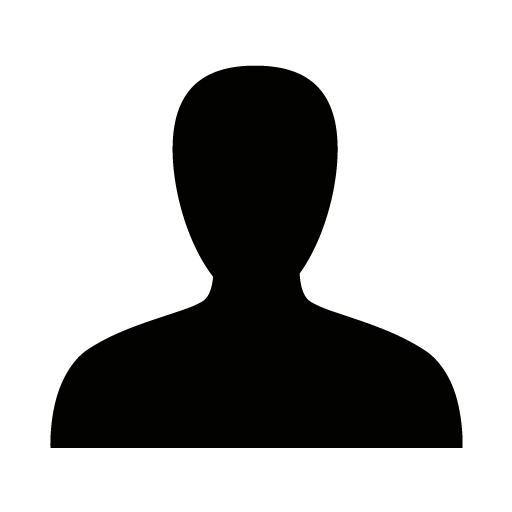
Lithium (Li) transition metal (TM) oxides composed of earth-abundant TMs, such as manganese (Mn), are a promising class of Li battery cathode materials with high energy density that can alleviate potential TM supply chain bottlenecks in battery manufacturing [1, 2]. Fundamental challenges in Mn-rich cathodes arise from phenomena such as large structural changes due to collective Jahn-Teller distortion of Mn3+, Mn migration, and phase transformations to spinel-like order, all of which impact the electrochemical performance [1, 3]. These physically complex phenomena motivate our ab initio re-examination of the Li-Mn-O rock salt space.
In this presentation, we focus on the thermodynamic and structural properties of several low-energy LiMnO2 polymorphs, which include the orthorhombic (Pmmn), layered (C2/m), lithiated spinel (I4/amd), and a previously unreported LiMnO2 phase with 𝛾-LiFeO2-like order [1, 3, 4]. DFT ionic relaxations are performed using an extensive range of density functionals spanning the generalized gradient approximation (GGA), meta-GGA, and hybrid-GGA, with or without Hubbard corrections (on-site and inter-site). Across all functional forms, we find that the anti-ferromagnetic (AFM) super-exchange interaction has a remarkably large impact on the total energies and resulting phase stability trends of these polymorphs, in agreement with previous studies [5]. We identify and analyze the substantial changes in electronic structure and bonding that arise from different spin configurations. The experimentally observed phase stability trends can be reproduced when accurately describing electron exchange and the magnetic ground-state. By comparing the electronic structure from DFT and many-body theory, we show that even the most advanced functionals do not fully capture the complex electronic correlations of this phase space. The phase stability at finite temperature is also evaluated with harmonic phonon calculations. Our first-principles analysis elucidates the rich relationship between the phase stability, electronic structure, magnetic order, and ionic configuration of the LiMnO2 polymorphs. Our methodology and fundamental understanding of Li-Mn-O phases developed in this study can be further harnessed to investigate compositionally similar, more recently developed Li TM oxide cathodes that are derived from this phase space.
Gielgud-O3
The ongoing development of solid electrolytes (SEs) for Li ion batteries has pushed their room temperature ionic conductivities to 1 mS/cm or beyond, bringing the realization of all-solid-state batteries within reach. All-solid-state batteries do not only promise improved safety compared to conventional batteries utilizing flammable liquid electrolytes. Moreover, it is currently investigated if the usage of SEs can sufficiently suppress dendrite growth, which might facilitate Li metal anodes and largely increase the energy density of the battery compared to conventional graphite anodes.
However, there are several issues that still need to be solved. One of these issues is the resistance due to the interface between SE and Li metal anode (SE|Li interface) that keeps rising with increasing charge-discharge cycles. One explanation for this observation is the formation of Li pores during the discharge process: The presence of pores leads to less interface area between SE and Li metal, leading to increased local current densities with high overpotentials. In this context, several studies have argued that one reason for the formation of pores is the low diffusion coefficient of Li atoms in the anode, unable to refill created vacancies at the interface towards the solid electrolyte fast enough.
In this contribution we will put the aforementioned argument to a test. To this end, we present results obtained from kinetic Monte Carlo simulations, in which we have modeled the Li metal anode connected to a virtual SE during the discharge process. By varying the externally applied current density and the interaction strength between SE and Li metal, we show two things: First, the diffusive species of interest for this process are not Li metal atoms, but rather Li vacancies. Second, we find that the SE|Li interface thermodynamics plays a much more crucial role in the kinetics of pore formation than the blamed diffusion coefficients in the metal. Therefore, we conclude that a careful preparation of SE|Li interfaces, potentially by means surface treatments or coatings of the solid electrolyte, are necessary to enable high power densities for all-solid-state batteries employing Li metal anodes. Furthermore, these insights are transferable to Na system and highlight the importance of interface engineering in all-solid-state batteries.
Gielgud-O4
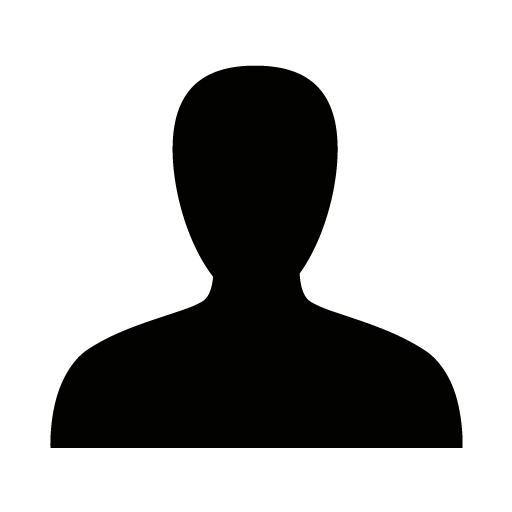
Magnesium (Mg)-batteries, which form a subset of multivalent (MV) electrochemical system, are a promising alternative to state-of-the-art lithium ion batteries (LIBs) due to their high theoretical volumetric energy density, natural abundance of Mg, safety, and low cost. However, the need for a high-voltage intercalation cathode with high-enough Mg-ion mobility remain a bottleneck for the realization of practical Mg-batteries. The poor mobility of Mg2+ in ionic solids is often attributed to stronger local electrostatic interactions that cause larger fluctuations in the underlying potential energy surface, resulting in higher migration barriers (Em). Thus, if the potential energy surface is flattened, via suitably modifying the underlying structure and/or the local coordination environment of Mg, the Em can be lowered and Mg motion can be made facile. Thus, in this work, we use a combination of density functional theory (DFT), machine learned interatomic potentials (MLIPs), and molecular dynamics (MD) to evaluate the Mg intercalation thermodynamics and Mg-transport properties of amorphous oxides as potential Mg/MV cathodes. Specifically, we generated a DFT-calculated dataset based on vanadium oxides and trained a moment tensor potential (i.e., an MLIP) to model large supercells mimicking an amorphous framework over longer time scales. We use pair distribution functions to verify the creation of an amorphous structure and active learning to ensure that our MLIP model has trained successfully. Subsequently, we find that the amorphous structure can indeed enhance Mg motion compared to the crystalline framework, with a loss of ~0.2 V in average Mg intercalation voltage over the entire Mg composition range. Our work demonstrates that computational frameworks can be used for unearthing novel amorphous Mg cathode materials, which can eventually result in the construction of a practical MV battery.
Gielgud-O5
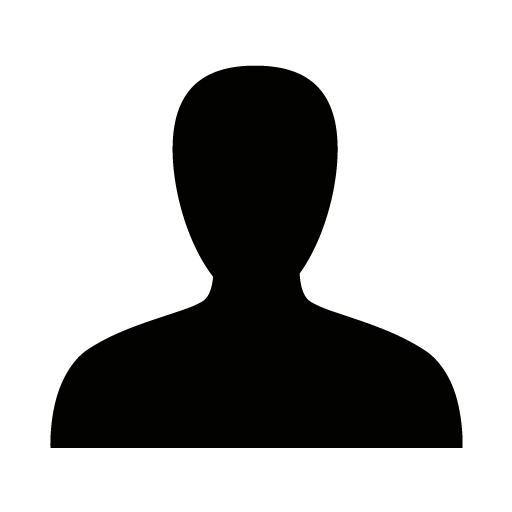
Overcoming the challenge of limited data availability within materials science is crucial for the broad-based applicability of machine learning within materials science, specifically the well-established deep learning paradigms that have been successful in image and natural language processing. One pathway to overcome this limited data availability is to use the framework of transfer learning, where a pre-trained machine learning model (on a larger dataset) can be fine-tuned on a target (typically smaller) dataset. Our study explores the utility of transfer learning as a systematic solution to address the lack of large datasets in materials science. We focus on developing a framework that leverages a Graph Neural Networks (GNN) to transfer learn material properties. To achieve this, we used seven diverse curated datasets from MatBench, encompassing sizes ranging from 941 to 132,752 data points. These datasets cover a spectrum of material properties, ranging from band gaps, to formation energies, and piezoelectric moduli. We employed three distinct pre-training strategies primarily to capture the impact of dataset size. Subsequently, we fine-tuned each pre-trained model on the remaining six datasets, once again employing three fine-tuning strategies to determine best performance. Importantly, we find that GNNs with greater flexibility during fine-tuning exhibited higher performance (in terms of r2scores), even surpassing models constructed from scratch using the respective datasets. Furthermore, we also examine the utility of our pre-training and fine-tuning strategies on properties that had minimal physical correlation with the seven pre-training datasets considered. In summary, we demonstrate transfer learning as a pivotal strategy by facilitating a comprehensive understanding of material properties, bridging data gaps, and enhancing material property prediction.
Gielgud-O6
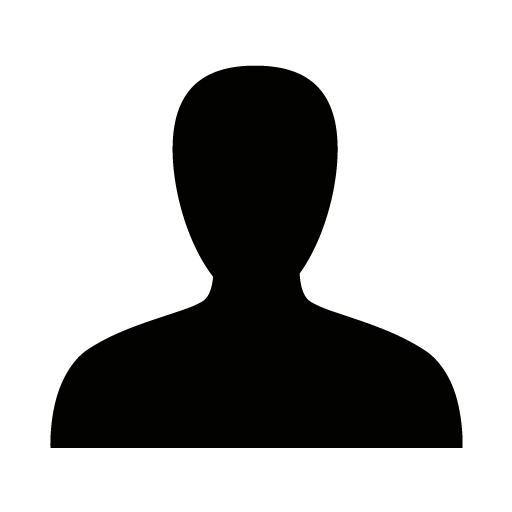
Calcium-ion batteries (CIBs) are under investigation as next-generation energy storage devices, offering a promising alternative to lithium-ion batteries due to their theoretically high energy density, better safety and lower costs associated with the natural abundance of calcium. However, the limited availability of positive electrode (cathode) materials has constrained the development of CIBs, and the pressing challenge lies in identifying cathode materials capable of reversible Ca2+ intercalation with optimal energy density and power performance. In this presentation, I will present our effort in addressing this challenge by using first-principles calculations to explore the chemical space of 3d transition metal fluorides (where transition metal can be from Ti to Ni). Specifically, we will look at fluorides adopting the weberite and perovskite structures as potential Ca-cathodes. Our study systematically evaluates key cathode properties, including ground state structure, average intercalation voltage, thermodynamic stability (at 0 K), and Ca2+ migration barriers. Importantly, we identify Cr- and Mn-based structures to be promising based on our calculated data. We not only unveil potential Ca-cathodes but also pave the way for further advancement in transition metal fluoride-based intercalation Ca-cathodes, expanding the chemical space for next-generation Ca-based energy storage technology.
Fleming-T2
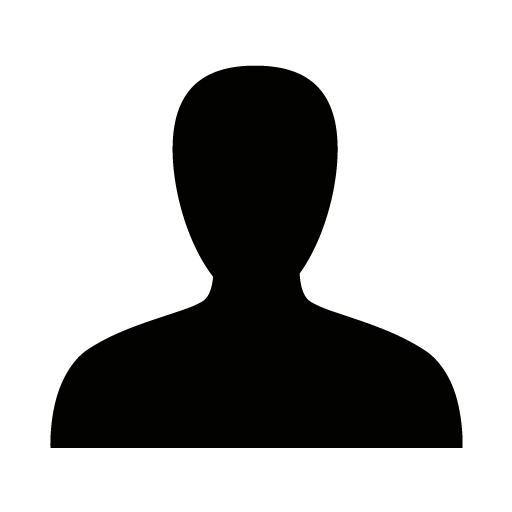
Starting out from the consideration of ionic charge carrier distribution at interfaces, “nanoionics” has developed into an established field within Solid State Ionics, covering not only conductivity aspects but also storage phenomena. Even though nanoionics is already relevant for interfacial phenomena in semi- infinite systems, it unfolds its significance most pronouncedly in mesoscopic systems where interfaces are so narrowly spaced that their impact is perceived at any position.
The history of the field is set out in terms of examples ranging from Heterogeneous Electrolytes to Job-
Sharing Storage and Bio-Ionics [1].
Starting out from the consideration of ionic charge carrier distribution at interfaces, “nanoionics” has developed into an established field within Solid State Ionics, covering not only conductivity aspects but also storage phenomena. Even though nanoionics is already relevant for interfacial phenomena in semi- infinite systems, it unfolds its significance most pronouncedly in mesoscopic systems where interfaces are so narrowly spaced that their impact is perceived at any position.
The history of the field is set out in terms of examples ranging from Heterogeneous Electrolytes to Job-
Sharing Storage and Bio-Ionics [1].
[1] J. Maier, Physical Chemistry of Ionic Materials. Ions and Electrons 2nd Edition, Wiley, 2023.
Fleming-T1
Oxygen ion conductors, both ionic and mixed conductors, are critical materials for the development of the important devices needed to achieve net-zero. These devices include the solid oxide fuel cell, solid oxide electrolyser cells, gas sensors and oxygen transport membranes. Unfortunately, the oxygen ion (O2-) is the largest ion in oxide lattices with an ionic radius of ~1.4 Å compared to, for example, a zirconium ion (Zr4+) at ~0.7Å . Student texts show how, by close packing the oxygen sublattice, various oxide structures can be achieved by populating the interstices with the appropriate, and usually much smaller cations. Thus, in principle, moving oxygen ions through the oxide lattice is a difficult process. Atomic transport does occur however because of the presence of crystal imperfections, i.e. point and extended defects present through either intrinsic or extrinsic processes.
this contribution I will review the development of ceramic oxygen ion conductors starting with a review of the history of these materials, the present state of the art and then some pointers to future development. Particular focus will be on the fluorite structured electrolyte materials and the mixed conducting perovskite and perovskite related materials. At elevated temperatures lattice oxygen transport can reach quite extraordinary levels for the solid state, with tracer diffusion coefficients approaching ~ 10-6cm2 sec-1 at close to 1273K. An important question is can this be high oxygen transport level be bettered by novel materials approaches such as high entropy oxides?
Because of the polycrystalline nature of practical devices, the oxygen ion must also cross the many interfaces in the device such as the gas/solid interface, for oxygen reduction and evolution, homogeneous solid/solid interfaces, i.e. grain boundaries in the ceramic components and heterogeneous interfaces e.g electrode/electrolyte interfaces. All these interfaces need to be optimised for ion transport and remain optimised for the operating lifetime of the device. To achieve this goal, we need to take a more holistic view of ion transport in these materials and not only focus on the most mobile species, but look at the slower process that can dominate in both processing and during the long timescales needed for commercial operation.
Fleming-K1
Diversification of battery technologies is crucial both to reduce dependence of specific raw materials and, most important, to adapt to specific use requirements including not only performance figures of merit (energy density, power, lifetime etc.,) but also economic and environmental considerations. Among the different possible rechargeable battery concepts, those based on multivalent charge carrier ions, such as Mg2+, Ca2+, Zn2+ or Al3+, have attracted great attention, especially coupled to the use of the corresponding metal as negative electrode. Proof-of-concept for Mg batteries was achieved already in 2000 while exploration of the analogous systems is much more recent. None of such concepts has yet reached the market, as significant hurdles remain, affecting not only electrolyte and negative electrode (efficiency of plating/stripping process) but also the positive electrode. In this regard, migration of multivalent charge carrier ions in inorganic hosts has been proved to be sluggish due to strong coulombic interactions which can be diminished by enhancing the covalency of the bonding and moving from oxides to sulfides, which penalizes the operation potential.
Open framework structures have also recently attracted attention, amongst which Prussian Blue analogues (PBAs) AxM[M’(CN)6]y · zH2O (A = alkaline metal (mostly K+ or Na+); M and M’ = transition metals; 0 ≤ x ≤ 2; y ≤ 1) represent an interesting alternative, which has proved excellent performances in Na and K based batteries. Their structure oconsists of a double perovskite framework with (C≡N)− anions bridging MN6 and M’C6 octahedra. A+ and H2O occupy the cubes defined by the transition metal framework. These have shown electrochemical activity in aqueous electrolytes containing multivalent cations but controversies remain as to whether the redox response observed being related to the intercalation of protons rather than divalent ions.
The study of these compounds in non-aqueous electrolytes is appealing, especially if both M and M’ are redox active (e.g. Fe, Mn), and they have shown excellent performance in K+ and Na+ batteries with very good kinetics and cycle life. The presentation will deal with analogous studies carried out in Mg2+ and Ca2+ containing electrolytes. Electrochemical performance, possible side reactions, and operando diffraction studies aimed at unravelling the redox mechanism will be discussed.
Fleming-K2
The success of rechargeable lithium-ion batteries (LIBs) has brought evident convenience to human society, but state-of-the-art LIBs with a graphite anode are approaching their energy density limits. Li metal is considered the ultimate anode material due to its ultra-high theoretical specific capacity of 3860 mAh g-1, which is more than 10 times higher than lithiated graphite. Nonetheless, Li metal anode suffers from poor safety and low cycling efficiency due to its high reactivity. Electrolytes that work with Li anode should possess excellent stability against Li metal or form a highly passivating interface. It is also critical to control the amount of Li in the cell, preferably having no excess Li at the anode side. In this presentation, next-generation electrolytes that enable such types of high-performance Li metal batteries will be discussed, including advanced garnet-type ceramic electrolytes and hybrid electrolytes. Also, recent progress in the development of solid state sodium ion electrolytes based on silicate-structure and solid state polymer electrolytes for solid state Na batteries will be presented.
Fleming-O1
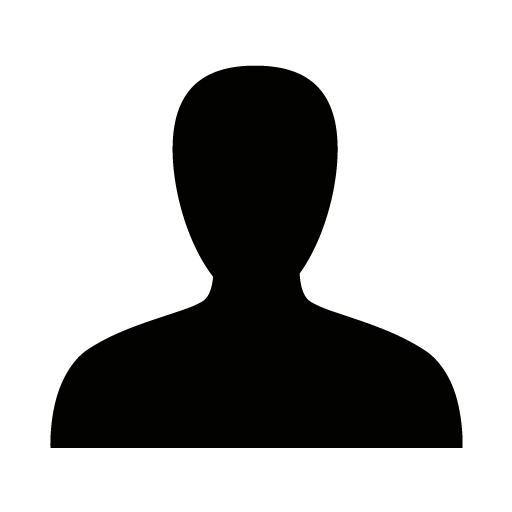
Magnesium batteries have been considered as sustainable energy-storage solutions beyond lithium-ion batteries due to the potential advantages of the Mg metal anode, such as high Mg earth abundance, low costs and high theoretical volumetric energy density.[1] To circumvent the drawbacks of passivation and corrosion of the Mg metal anode, and ensure higher levels of safety, solid-state battery concepts are being pursued. Nevertheless, the high charge density of the Mg2+-ion, compared to the Li+/Na+ counterparts, leads to strong coulombic interactions in the solid host-framework, making the development of suitable Mg-ion conductors quite challenging.
Among the potential candidates for inorganic Mg-ion solid electrolytes, the crystal structure of MgB2X4 spinels offers a relatively large distance between the B-cations and the mobile Mg-cation in its trigonal transition state along the tetrahedra-octahedra-tetrahedra Mg-ion migration path.[2] This results in inherently weaker cation-cation repulsion, possibly crucial to enabling multivalent-ion conduction even at ambient temperature. Moreover, computational investigations on the magnesium chalcogenides MgB2X4 (B = Sc, Er, Tm, Y; X = S, Se) predict low Mg2+ migration barriers (<415 meV), making these materials to be potential candidates as good Mg-ion conductors.[3,4] Especially, the compounds with larger B-ions (Er, Tm, Y) are likely more promising, since the shared trigonal face between tetrahedra and octahedra along the migration path is widened and therefore higher ionic conductivities can be expected. However, with exception of a handful practical work on MgSc2Se4,[4-5] no experimental conductivity studies on the other magnesium chalcogenide spinels exist, and an unequivocal electrochemical evidence for the Mg-ion transport in these materials is still missing.[6]
To address this challenge, we successfully synthesized five of the MgB2X4 spinels (B = Sc, Er, Tm, Y; X = S, Se) via solid-state reaction. The phase-purity of the prepared compounds was examined by X-ray powder diffraction combined with Rietveld refinements. DC polarization and electrochemical impedance measurements were performed to investigate the electronic partial conductivity. Additionally, we present the first electrochemical evidence for Mg-ion transport in the spinels using two independent methods, namely reversible Mg plating/stripping cycling and electrochemical deposition of Mg metal. To overcome the difficulty of measuring the ionic conductivity caused by the known mixed-conducting character, we inserted two pure ion-conducting interlayers in a symmetrical transference cell. Thus, the electron transport was effectively suppressed, allowing an accurate determination of the room-temperature ionic conductivities (5.5x10–6-6.5x10–5 S cm–1) and the low Mg-ion migration barriers (426-381 meV) of the MgB2X4 spinels. These findings demonstrate the potential of the magnesium chalcogenide spinels as a promising class of solid electrolytes and open a new door for exploring additional mixed-conducting Mg-based phases.
Fleming-O2
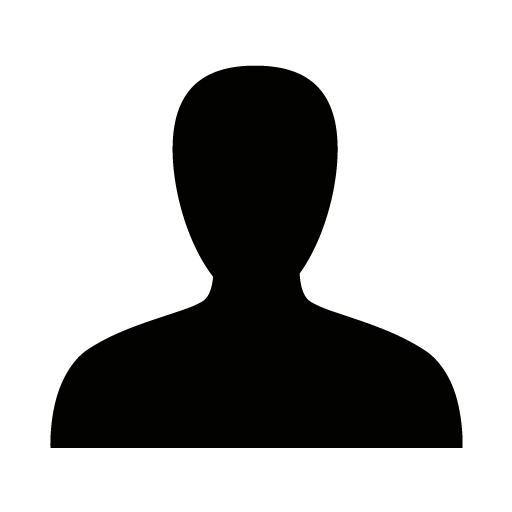
The development of solid state battery has been witnessed an increasing demand owing to the merits of solid electrolytes, including the intrinsic safety to avoid thermal runaway, the superior mechanical properties to suppress bidirectional crosstalk inside the batteries, and the high transference number to eliminate the concentration polarization at high current densities.[1] Apart from the lithium battery technologies, magnesium batteries using multivalent cations as charge carriers are also of great research interest due to the high earth abundance of magnesium source, and high theoretical energy density given by multi-electron redox reactions.[2] Nevertheless, the large charge density of Mg2+ cations inevitably causes sluggish kinetics of the ion migration, compared to the lithium counterpart.[3] A solid electrolyte material with high ionic conductivity, is thus the prerequisite for the development of solid state Mg batteries. In this study, we prepared a new Mg-ion conducting NASICON-structured material, Mg0.5Sn2(PO4)3.[4] By combining it with a small amount of Mg ionic liquid to improve the Mg2+ migration at grain boundary, the prepared Mg-ion conducting hybrid solid electrolyte shows superior room-temperature ionic conductivity of 1.1 · 10–4 S cm–1 and an activation energy of 0.36 eV. Reversible Mg plating/stripping is realized at room temperature with stable cycling performance. Surface analysis demonstrates a stable interface between the electrolyte and Mg metal anode, suggesting good compatibility. Using in situ electrochemical scanning electron microscopy, for the first time we observed the room temperature Mg growth inside a solid-state cell by the evidence of clear-cut metal particle formation and electrolyte particle cracking. The key parameters that affect the Mg metal deposition properties are also explored. The results shown here can act as a good starting point for the understanding of Mg transport behavior in solid-state batteries.
Fleming-O3
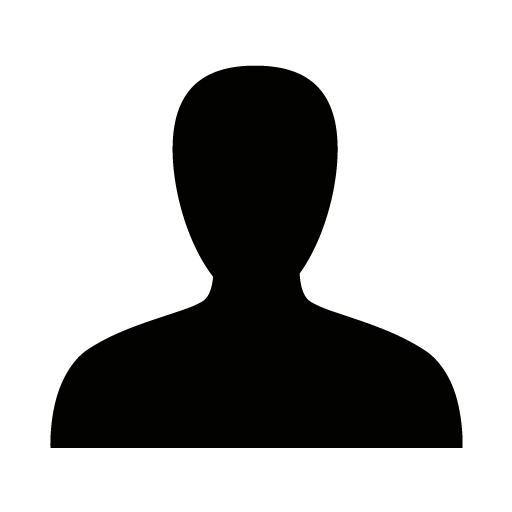
Solid-state batteries are expected to be next-generation batteries in the low carbon society due to their high reliability. Garnet-type solid electrolytes are regarded as a promising electrolyte for the batteries because of their conductivities are as high as 10-3 S cm-1 and their stability against lithium metal. Although lithium metal anodes will provide high energy densities to the batteries, lithium metal anodes on garnet-type solid electrolytes often exhibit dendritic growth during charging leading to internal short-circuit.
The critical current density for the dendritic growth will strongly depends on the surface chemistry of garnet. For example, exposure of garnet to ambient air forms a contamination layer of Li2CO3 and LiOH with protonation of the garnet. Since the contamination layer is a poor ionic conductor, it impedes lithium-ion transfer at the interface to the electrode. Therefore, the charging current is concentrated to part of the Li/garnet interface where the contamination layer is not formed, resulting in the short circuit. Various methods to remove the contamination layer have been proposed in order to suppress the internal short-circuit: surface polishing, heat treatment to react Li2CO3 with protonated garnet, and that reacting the Li2CO3 with carbon; however, their effects are limited. We will present a very simple method to remove the contamination layer and effectively suppress the internal short-circuit at the conference.
Fleming-O4
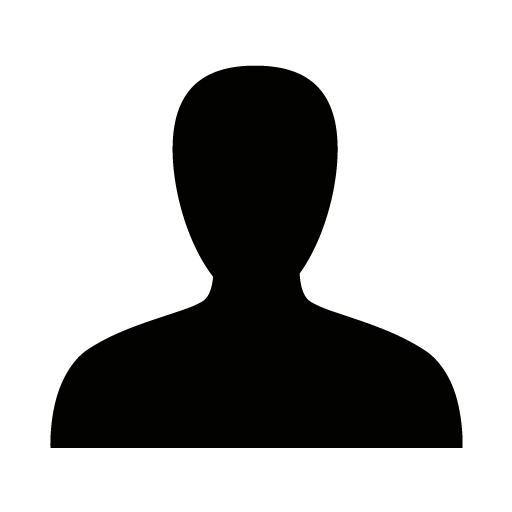
Perovskite-type solid electrolytes have attracted attention as model materials for solid-state battery materials due to their high ionic conductivity and simple structure. A perovskite, La0.57Li0.29TiO3 (LLTO), shows ionic conductivity of 1×10-3 Scm-1 at room temperature. It has a double perovskite structure consisting of a La-rich layer in which La occupies 96% of the A site and a La-poor layer in which La occupies 23% of the A site. Due to its crystal structure, anisotropic lithium conduction, in which lithium ions migrate through the La-poor layer, is expected, but anisotropic ionic conduction is rarely observed even in LLTO single crystals [1, 2]. This is believed to be due to the presence of 90° domains. In addition, the complex hierarchical structure, including 90° domains and grain boundaries, makes it difficult to separate bulk and grain boundary diffusion.
This study focuses on the use of pulsed field gradient nuclear magnetic resonance (PFG-NMR) spectroscopy to elucidate the bulk diffusion mechanism of LLTO. Sample is a commercially available LLTO (Toho Titanium) polycrystalline ceramic. The microstructure of the polycrystals was characterized by SEM, EBSD, and STEM. LLTO polycrystals are consisted of random grains (10-200 μm) and 90° domains (10-100 nm). PFG-NMR were performed using an ECA-400 NMR system with a diffusion probe in the temperature range of 273K to 393K. An ECA-500 spectrometer and a high temperature diffusion probe [3] were used for measurements at higher temperatures. A pulsed gradient-guided echo sequence was used. Typical diffusion time Δ is 10-100 ms [4].
In the case of homogeneous diffusion, the echo attenuation of PFG-NMR follows the Stejskal-Tanner equation and decays linearly with the square of the intensity of the gradient pulse (g). In LLTO, however, the decay is not linear but curvilinear. This curve fits very well in the theoretical equation for randomly oriented two-dimensional crystals, providing evidence for two-dimensional diffusion of LLTO. We found that at 393 K, the diffusion coefficient of LLTO is 6.1 × 10-7 cm2 s-1 (ab-axis) and 3.3 × 10-8 cm2 s-1 (c-axis). This diffusion anisotropy was maintained up to 693 K, the highest temperature measured. On the atomic scale, it is suggested that the diffusion path of LLTO may change at high temperatures [5], but on the millisecond scale, the macroscopic anisotropy does not appear to change.
The average diffusion coefficient (DNMR) is 2/3 of the diffusion coefficient for the ab direction. When compared to the conductivity diffusion coefficient (Dσ) obtained from the bulk ionic conductivity, it agrees well over a wide temperature range in an Arrhenius plot. The ionic conductivity is also an average of the anisotropic conductivity of the LLTO. Non-Arrhenius behavior is observed above 450 K for both DNMR and Dσ. The origin of the non-Arrhenius behavior is not due to a change in the number of carriers, but to a change in the mobility. In addition, the grain boundary diffusion coefficient can be elucidated by tracer isotope diffusion using secondary ion mass spectrometry (SIMS) [6]. In conclusion, PFG-NMR is a promising method for elucidating the diffusion mechanism of polycrystalline solid electrolytes.
Westminster-K1
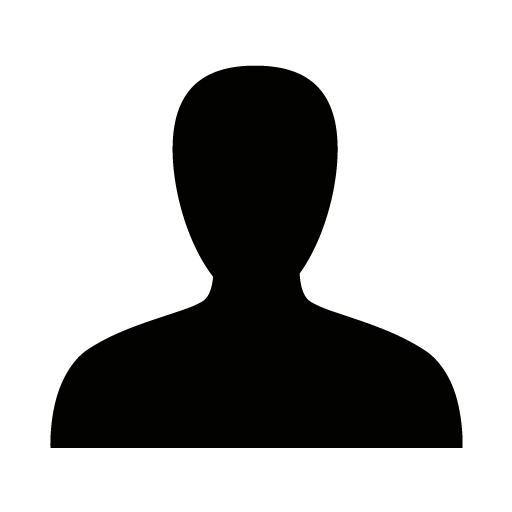
Energy storage is critical to achieve ambitious targets for Net Zero where Li-ion and post Li-ion chemistries will be a cornerstone of decarbonisation efforts in a range of sectors.
To support the acceleration of energy storage technologies, the past decade has seen the rapid development and proliferation of three-dimensional X-ray imaging tools applied to batter materials and devices This provides a framework to improve the understanding of electrode morphology and its influence on transport processes, electrochemistry and mechanical behaviour. Moreover, through the application of image-based modelling tools, it has become possible to simulate a range of phenomena using a computational framework derived directly from tomographic images.
The non-destructive, and multi-scale characteristics of X-ray imaging tools provide benefits to quantify hierarchical complexity from the particle to the electrode and device level, where the portfolio of techniques available includes: absorption and phase contrast CT to understand multi-scale morphology; XRD-CT to reconcile chemical, crystallographic and morphological behaviour and, Bragg Coherent Diffraction Imaging to access sub-particle behaviour. Meanwhile complementary neutron, electron and ion beam imaging techniques can also leverage the benefits of the alternative contrast modes possible.
Here, we will review this progress and reflect on recent developments using multi-modal methods to understand the performance of advanced batteries. In concert, the portfolio of imaging and modelling tools provides a platform to explore the performance, degradation and failure of Li-ion batteries and to accelerate the development of post Li-ion chemistries.
Westminster-I1
Battery companies want to know the relationship between their manufacturing parameters and the performance of the resulting cells, so that they can optimise their products for particular applications, reduce costs, and improve yield. The literature contains many examples of physics-based models of the various manufacturing processes (including mixing, coating, drying and calendaring), but these systems are hugely complex, and as a result they are expensive to simulate and hard to validate.
Recent advances in generative machine learning (ML) methods have allowed the relationship from manufacturing parameters to microstructure to be directly learned from data.
In this talk I will present a modular approach to electrode microstructural optimisation cycle that makes use of these ML methods, in combination with GPU accelerated metric extraction (TauFactor 2), electrochemical cell simulation (PyBaMM), and Bayesian optimisation. We are always looking for new collaborations and new data so please get in touch! If you’d like to use any of our suite of open-source tools, then head to our website: https://tldr-group.github.io
We’ve also just spun-out a company from Imperial, called Polaron AI, to bring these tools to market. Check out our website (www.polaron.ai) and get in touch: info@polaron.ai
Westminster-O1
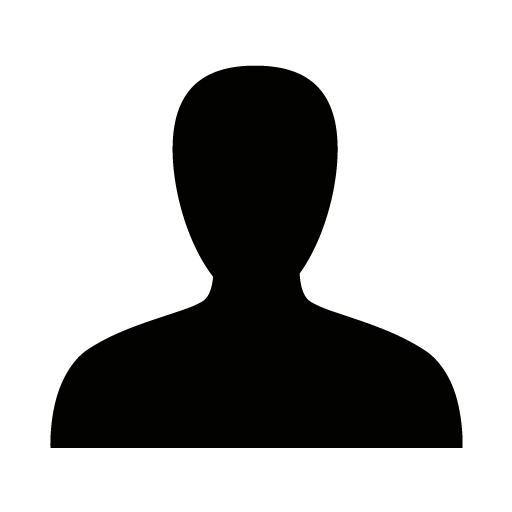
Efficient synthesis recipes are needed both to streamline the manufacturing of complex materials and to accelerate the realization of theoretically predicted materials. Oftentimes the solid-state synthesis of multicomponent oxides is impeded by undesired byproduct phases, which can kinetically trap reactions in an incomplete non-equilibrium state. We present a thermodynamic strategy to navigate high-dimensional phase diagrams in search of precursors that circumvent low-energy competing byproducts, while maximizing the reaction energy to drive fast phase transformation kinetics. Using a robotic inorganic materials synthesis laboratory, we perform a large-scale experimental validation of our precursor selection principles. For a set of 35 target quaternary oxides with chemistries representative of intercalation battery cathodes and solid-state electrolytes, we perform 224 reactions spanning 27 elements with 28 unique precursors. Our predicted precursors frequently yield target materials with higher phase purity than when starting from traditional precursors. Robotic laboratories offer an exciting new platform for data-driven experimental science, from which we can develop new insights into materials synthesis for both robot and human chemists.
J. Chen, S. R. Cross, L. J. Miara, J-J Cho, Y. Wang, W. Sun, Nature Synthesis (2024, in press)
Westminster-O2
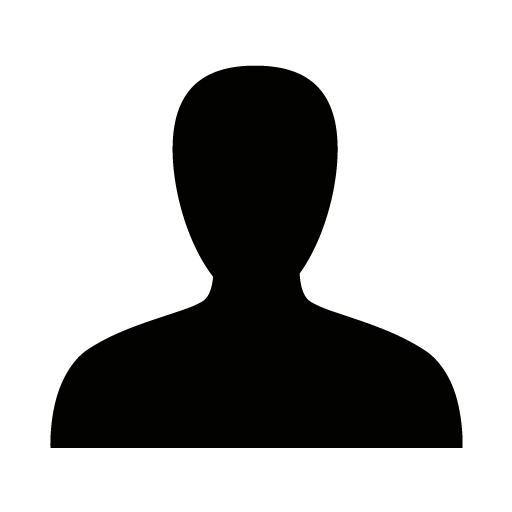
The topotactic phase transition of some perovskites, from perovskite ABO3−δ to brownmillerite A2B2O5, has recently attracted attention because of its potential for application in redox-induced resistance random access memories (ReRAM). The perovskite phase with its disordered vacancy distribution shows high oxide-ion mobility in contrast to the brownmillerite phase with its one-dimensionally ordered structural vacancies. The key process for the phase transition is the diffusion of oxygen point-defects, but oxygen diffusion coefficients have been obtained neither experimentally nor computationally. Furthermore, insights into the mechanisms of oxygen diffusion from atomistic simulations have been limited mainly to static calculations.
In our study, we employed molecular dynamics (MD) simulations with empirical pair potentials (i) to obtain oxygen diffusion coefficients for both the brownmillerite Sr2Fe2O5 phase and the perovskite SrFeO2.5 phase; and (ii) to elucidate the paths and mechanisms of oxygen diffusion. In particular, we investigated non-stoichiometric systems with oxygen excess or oxygen deficiency in order to study diffusion processes by means of oxygen interstitials or oxygen vacancies independently from each other. The investigation allowed us to achieve a deep and detailed description of diffusion processes in brownmillerite Sr2Fe2O5.
Westminster-O3
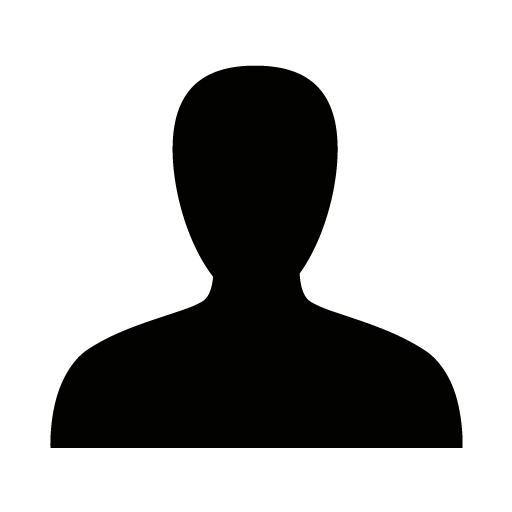
It is imperative to have highly conductive oxide ion and proton conductors that operate at intermediate temperatures to improve the performance of ceramic fuel cells. The crystal structures of these materials significantly influence their ionic conduction properties. Identifying novel materials with enhanced conductive abilities is a crucial research focus, promising substantial advancements in future fuel cell technologies.
In pursuing this goal, we focus on advanced perovskite solid electrolytes and their interfaces. Employing atomistic simulations, we aim to unravel the complexities of these materials, gaining a detailed understanding of ion transport mechanisms crucial for optimizing electrolyte performance and efficiency. Our approach integrates the predictive capabilities of density functional theory with the dynamic nature of molecular dynamics, supplemented by machine-learning based potentials for a thorough analysis.
To address larger system sizes and extended time scales in our modelling, we utilize machine learning potentials, specifically moment tensor potentials (MTPs) [1]. The crucial potential fitting process relies on energies, forces, and stresses obtained from diverse configurations through ab initio calculations (AIMD) [2]. Our study focuses on the systems Sr3V2O8 and Ba7Nb4MoO20, and our employed MTPs, fitted together with active learning, exhibit excellent agreement with data from AIMD. Notably, oxygen and protons' diffusion coefficients, along with oxygen ions' diffusivity, show commendable consistency with both AIMD simulations and experimental data. This alignment underscores the accuracy and reliability of our MTPs in capturing the intricate dynamics of these materials, reinforcing their suitability for modelling and predicting the behaviour of Sr3V2O8 and Ba7Nb4MoO20.
Westminster-O4
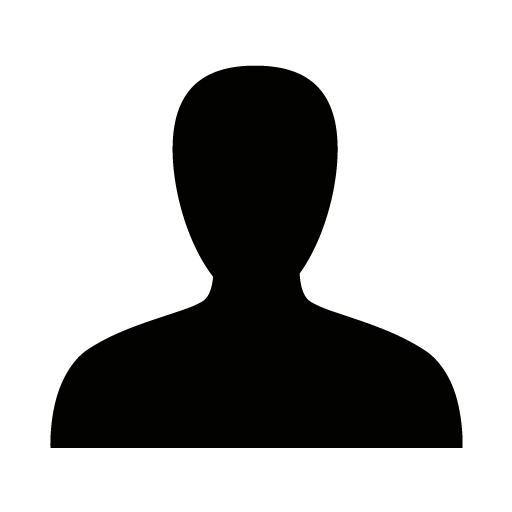
The atomistic structure of lithium nickelate (LiNiO2), the parent compound of Ni-rich layered oxide cathodes for Li-ion batteries, continues to elude a comprehensive understanding. In particular, the local structural environment and the role of Jahn-Teller distortions are unclear, as are the interplay of distortions and point defects, and their influence on cycling behaviour. Through a combination of ab initio molecular dynamics (AIMD) simulations and variable-temperature X-ray diffraction (VT-XRD), we explore Jahn-Teller distortions in LiNiO2 as a function of temperature.[1] Static Jahn-Teller distortions are observed at low temperatures (T < 250 K), followed by a broad phase transition occurring between 250 K and 350 K, leading to a highly dynamic, displacive phase at high temperatures (T > 350 K), which does not show the four short and two long bonds characteristic of local Jahn-Teller distortions. Between 250 K and 350 K, a mixed-phase regime is found via the AIMD simulations where distorted and undistorted domains coexist. The repeated change between the distorted and undistorted states in this mixed phase regime allows the Jahn-Teller long axes to change direction, these pseudorotations of the Ni-O long axes being a side effect of the onset of the displacive phase transition. Antisite defects, involving Li ions in the Ni layer and Ni ions in the Li layer, are found to pin the undistorted domains at low temperatures, impeding cooperative ordering at a longer length scale. Using 7Li NMR measurements in combination with density functional theory (DFT) calculations, we predict the 7Li Fermi contact shifts for the Jahn-Teller distorted and undistorted structures, the experimental 7Li room temperature spectrum being ascribed to an appropriately weighted time-average of the rapidly fluctuating structure comprising, collinear, zigzag and undistorted domains. The 7Li NMR spectra are sensitive to the nature and distribution of anti-site defects, and in combination with DFT calculations we identify 7Li resonances characteristic of specific antisite-defect configurations.
St.James-K1
Dr. Jeff Sakamoto is a Professor in the departments of materials and mechanical engineering at the University of California, Santa Barbara where holds a Mehrabian Endowed Chancellor's Chair appointment. He recently moved from the mechanical engineering and materials science and engineering departments at the University of Michigan (UM). He is also the Director of the US Department of Energy, Energy Frontier Research Center, Mechano-chemical Understanding of Solid-Ion Conductors (MUSIC). He investigates ceramic ion conductors focusing on mechano-chemical phenomena in applications such as lithium and sodium metal solid-state batteries and flow cells for grid storage. He is also involved in the UM EV Center and second generation UM Battery Lab. Professor Sakamoto has 25 years of experience conducting fundamental, applied, and translational research on materials for terrestrial and space energy technologies. Before joining UM, he was an Assistant Professor at Michigan State University (2007-2014) and a senior engineer at the Caltech Jet Propulsion Laboratory (2000-2007). At the Jet Propulsion Laboratory he helped develop Li ion batteries for the 2003 Mars rovers. He is the founder and CTO of the University of Michigan solid-state battery Startup Zakuro, Inc. He also received two Major Space Act Awards from the NASA Inventions and Contributions Board, is a Kavli Fellow, is the primary contributor on 18 granted and 21 pending patents, and received the Teacher-Scholar (2013), and Withrow Excellence in Teaching (2009) Awards at Michigan State University. Dr. Jeff Sakamoto is a Professor of Mechanical Engineering and Materials Science and Engineering at the University of Michigan (UM). He is also the Director of the US Department of Energy, Energy Frontier Research Center, Mechano-chemical Understanding of Solid-Ion Conductors (MUSIC). He investigates ceramic ion conductors focusing on mechano-chemical phenomena in applications such as lithium and sodium metal solid-state batteries and flow cells for grid storage. He is also involved in the UM EV Center and second generation UM Battery Lab. Professor Sakamoto has 25 years of experience conducting fundamental, applied, and translational research on materials for terrestrial and space energy technologies. Before joining UM, he was an Assistant Professor at Michigan State University (2007-2014) and a senior engineer at the Caltech Jet Propulsion Laboratory (2000-2007). At the Jet Propulsion Laboratory he helped develop Li ion batteries for the 2003 Mars rovers. He is the founder and CTO of the University of Michigan solid-state battery Startup Zakuro, Inc. He also received two Major Space Act Awards from the NASA Inventions and Contributions Board, is a Kavli Fellow, is the primary contributor on 18 granted and 21 pending patents, and received the Teacher-Scholar (2013), and Withrow Excellence in Teaching (2009) Awards at Michigan State University.
The recent emergence and discovery of new ceramic ion conductors (CICs) with fast ionic conductivity at near-ambient temperatures creates the opportunity to push the frontiers of electrochemical energy conversion and storage. The ability to replace traditional liquid or polymer electrolytes with ceramics has the disruptive potential to improve safety and enable next generation technologies including solid-state batteries with metal anodes, impermeable membranes to prevent crossover in redox flow batteries for long-duration energy storage (LDES), and intermediate temperature solid-oxide fuel cells to propel the hydrogen economy. Enabling the next generation of electrochemical conversion and storage, however, requires fundamental research to understand and control the emergent mechano-chemical environments that arise when CIC materials are interfaced with other dissimilar materials.
The United States Department of Energy is supporting the collaborative and interdisciplinary project Mechano-chemical Understanding of Solid Ion Conductors (MUSIC). The overarching scientific mission of MUSIC is to reveal, understand, and model, and ultimately control the chemo-mechanical phenomena underlying the processing and electrochemical dynamics of CICs for clean energy systems. This presentation will consist of highlights from MUSIC to include topics such as stress corrosion cracking, Li/Na free manufacturing, and composite cathode analyzed using complementary experimentation and computation.
St.James-I1
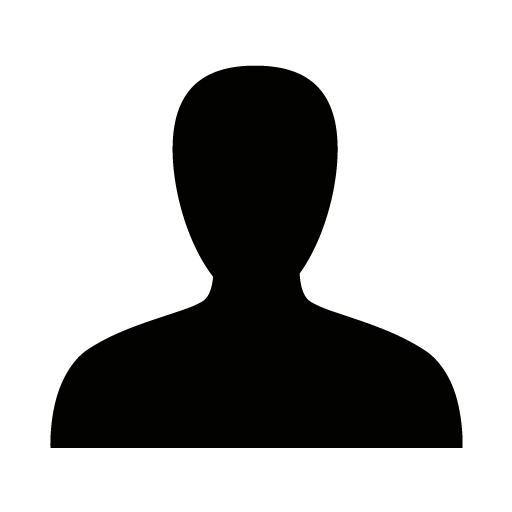
The functional properties of ceramics are usually tailored by designing point defects and interfaces. Dislocations as one-dimensional line defects are so far underrepresented means to modify respective properties. However, dislocations possess the ability to alter these extensively. Given new methods of introducing dense dislocation networks in multiple ceramics, extensive investigations found a significant impact on the conductivity of electronic and ionic oxide ceramic conductors. The opportunity to tune ceramics beyond what can be achieved by chemical doping is of significant interest. Further affected properties include ferroelectricity and photoelectric responses. The change in photoelectric and surface electrical behavior shows that dislocations can be a new pathway for altering important parameters concerning catalysis, photocatalysis, and modification of Schottky barriers. The impact of dislocations on the ceramics depends on a convolution of dislocation character, core properties, possibly existing space charges, and mesoscopic structure. It is thus essential to combine the understanding of mesoscopic dislocation structure and its mechanical behavior at elevated temperatures. This makes dislocations in ceramics an exciting interdisciplinary research field.
St.James-O1
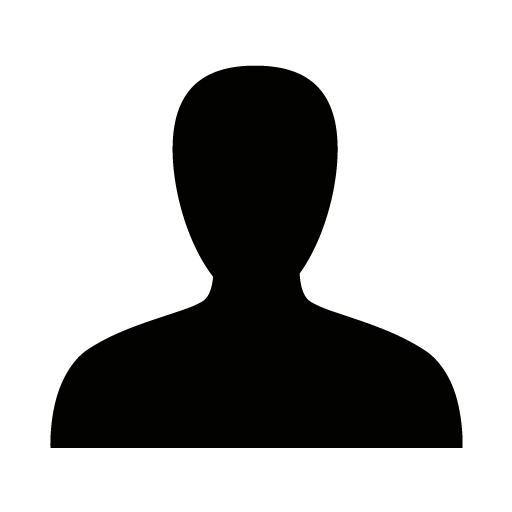
Overpotentials in porous electrodes lead to undesirable behavior during battery cycling and understanding the origins of these overpotentials is crucial. In Li-ion batteries, a significant fraction of the electrode overpotentials in layered cathodes arise from low-frequency sources. These low-frequency overpotential sources and the impedances they lead to are traditionally analyzed in the time domain using galvanostatic intermittent titration technique (GITT) or the frequency domain using electrochemical impedance spectroscopy (EIS). Currently in the standard porous electrode model, solid-state diffusion of Li in the cathode material is the only mechanism that is considered to lead to the electrochemical behavior seen at low frequencies and longer timescales. However, there are various experimental inconsistencies that emerge when attributing voltage relaxation behavior and low-frequency impedance behavior solely to solid-state Li diffusion. Thus, it is necessary to further investigate and understand the true nature of the impedances that arise in these timescale and frequency regimes.
In this work, we investigate the origins of the voltage relaxation behavior and low-frequency impedance in porous electrodes. Using a 3-electrode coin cell system, we vary the kinetics of the electrode/electrolyte interface by systematically changing the salt concentration of the electrolyte used. We perform GITT and EIS measurements and find that the relaxation behavior and low-frequency impedance changes as the kinetics of the electrode/electrolyte interface changes. These results contradict the experimental dependencies that are expected to arise from a system that is only diffusion controlled. Instead of a solid-state diffusion limitation, we propose an alternative mechanism that could also contribute to the observed GITT and EIS behavior: a distribution of reaction constants. We demonstrate the validity of this hypothesis with a multi-particle simulation. Furthermore, we characterize the hierarchical morphology of porous electrodes through particle physisorption and nano-computed tomography and investigate how their complex structures could lead to inherently broad distributions of electrochemical reaction times.
St.James-O2
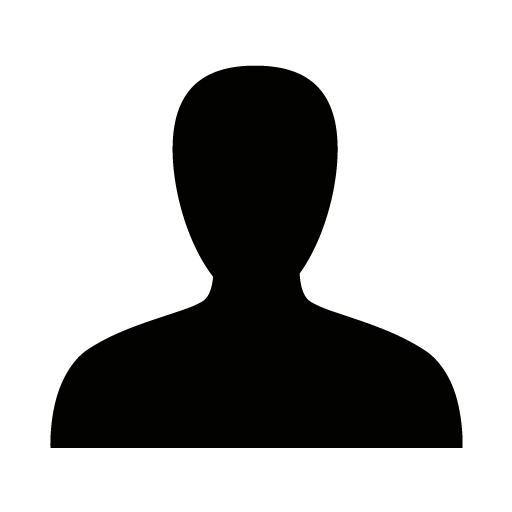
Carbon nanotubes (CNTs) have been studied in various fields (e.g., catalysts, sensors, and electrodes) due to their unique electronic and mechanical properties. Metal oxide/CNT composites, which combine the high theoretical capacity of metal oxides and the high conductivity of carbon materials, are attracting attention in the energy field such as batteries, supercapacitors, and fuel cells. CNTs can effectively anchor nano-sized oxide particles through a network structure of CNTs. The sp2 hybridization on the surface of CNTs can enhance the charge transfer between metal oxide particles and CNTs, providing high electrochemical activity.
Metal oxides/CNTs using noble metal oxides have high electrical conductivity and high chemical stability, and have been used as energy materials. However, due to their cost and scarcity, transition metal oxides such as NiO, Fe3O4, and MnO2 are being introduced recently. Nickel oxide (NiO) has high charge transfer properties owing to a reversible electron transfer reaction (Ni2+/Ni3+) involving the activated surface oxygen species or the metallic sites. To obtain the advantage of NiO, various synthesis methods are being studied for coating nano sized NiO particles on the surface of CNTs or embedding them inside CNTs.
We synthesized nano sized NiO/CNT by solution combustion synthesis to control the surface properties of NiO and investigated the electrochemical properties of NiO/CNTs by oxygen vacancy formation on the surface of NiO. In the crystal structure and particle size analysis, the lattice strain was confirmed as the diffraction peaks of NiO decreased and broadened. For the microstructure of NiO and the structural properties of CNTs, the CNT growth by nano sized NiO was observed, and the ID/IG ratio was calculated to be 0.36, indicating high graphitization of the CNT surface. The surface bonding state and valence state of NiO were analyzed, and EPR analysis was used to confirm the changes in oxygen vacancy formation on the NiO surface. G-factor was calculated, which show the formation of oxygen vacancies on the NiO surface by the enhanced charge transfer between NiO and CNT. Electrochemical properties of NIO/CNT will be discussed with and the charge transfer between NiO and CNT and surface oxygen vacancies by the changes in the valence state of NiO,
St.James-O3
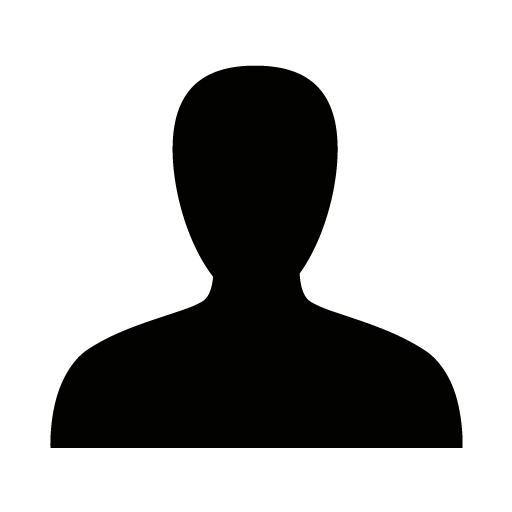
Developing solid electrolytes with high ionic conductivity, high Li-ion transference number (tLi+), and high electrochemical stability is strongly required for high-performance all-solid-state batteries. Molecular crystals have attracted considerable attention as a novel candidate for such solid electrolytes.[1] In this study, we fabricated the molecular crystalline electrolyte, Li2{N(SO2CF3)}2(NCCH2CH2CN)3 (Li2(TFSA)2(SN)3), through a quite simple process using Li{N(SO2CF3)2}2 (LiTFSA) and succinonitrile (SN) as precursor materials. Single crystal X-ray structural analysis confirmed the detailed crystal structure of Li2(TFSA)2(SN)3 with well-ordered arrangements of Li-ions corresponding to a Li-ion conduction path in the crystal lattice. Notably, Li2(TFSA)2(SN)3 demonstrated solid-state ion conductivity of 3 × 10-5 S/cm at room temperature, a high Li-ion transference number of 0.98, exceptional electrochemical stability for 5 V-class cathodes, and compatibility with the dissolution and deposition of Li metal. Additionally, scanning electron microscopy (SEM) observations revealed the presence of crystalline grain boundaries in this molecular crystalline electrolyte showing the significance of forming a crystal-based interface for selective Li-ion conduction within molecular crystalline electrolytes. These findings, based on the X-ray crystal structural determination, electrochemical measurements, and SEM observations, highlight the critical roles of component selection, conduction paths in the crystal structure, and crystalline interfaces in developing superior molecular crystalline electrolytes for advancing all-solid-state lithium batteries.
St.James-O4
I was trained in Chemistry at Imperial College London & ETH Zuerich with a focus on electrochemistry, materials science and computational chemistry. After obtaining my masters degree I did an internship on elecrtochemical CO2 reduction at the Fraunhaufer (IGB) Institute in Bavaria. Right after this, I started my PhD at the TU Delft in the Storage of Electrochemical Energy group where I investigate fully-reduced Li solid electrolytes combining experiments and ab-initio calculations. Fully-reduced electrolytes are thermodynamically stable against Li metal. In pratice this means that you can throw these electrolytes in molten Li metal (200 C) - no reaction would take place. Combining experiments and ab-initio calculations I developed novel, high-conducting solid electrolytes throughout my PhD that are thermodynamically stable against Li metal and thus "natural-born" candidates for anolytes facing low-potential electrodes such as Si or Li metal.
Most highly-conducting solid electrolytes decompose at the low operating potentials of next-generation anodes leading to irreversible lithium loss and increased cell resistance. Such performance losses due to electrochemical decomposition may be prevented by designing electrolytes which are thermodynamically stable at low operating potentials.
Here, we report on the discovery a new family of fully-reduced electrolytes by dissolving lithium nitride into the Li2S antifluorite structure, yielding highly conducting crystalline Li2+xS1-xNx phases, synthesized by mechanochemistry, identified by x-ray and neutron diffraction, and reaching high ionic conductivities (> 0.2 mS cm-1) at ambient temperatures. Combining impedance spectroscopy experiments and ab-initio density functional theory calculations we clarify the mechanism by which increased configurational entropy boosts ionic conductivity in Li2+xS1-xNx phases by five orders of magnitude compared to the Li2S host structure.
This advance is achieved through a novel theoretical framework, leveraging percolation analysis with local-environment-specific calculated activation barriers and is widely applicable to disordered solid electrolytes. Finally, we introduce the concept of “trapped” Li ions and how they may play an essential role when rationalizing changes in the Arrhenius prefactors from variable‑temperature conductivity measurements of disordered solid electrolytes. These findings pave the way to understanding the effect of disorder (and high-entropy) on ion-conduction in highly relevant disordered electrolyte and electrode battery materials. Additionally, we leverage the mechanistic insight developed here, to design a new class of highly-conducting solid electrolytes that could help eliminate decomposition‑induced Li losses on the anode side in high-performance next-generation batteries.
Moore-K1
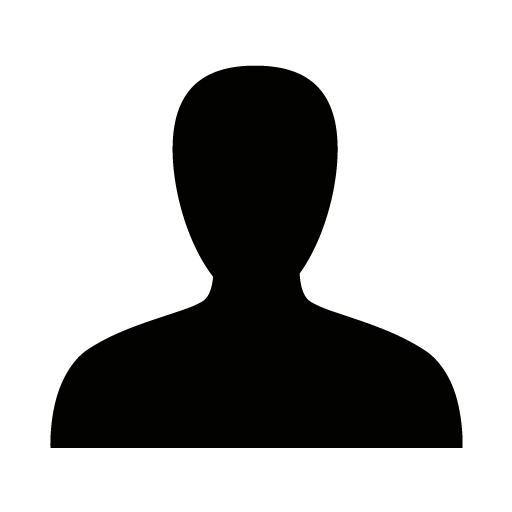
In this presentation, we will discuss La0.6Sr0.4Co0.2Fe0.8O3 (LSCF) – Ce0.9Gd0.1O2 (GDC) oxygen electrode stability during 100 to 12,000 hours solid oxide electrolysis cell (SOEC) operation. A systematic study was undertaken to understand potential phase evolution of LSCF in a broad range of experimental conditions, while varying sintering and operating temperatures, operating pressure, current, voltage as well as during dynamic SOEC operation and in the presence of Cr and S contaminants. Tests were performed using standard Ni-YSZ electrode-supported SOECs with a thin YSZ electrolyte. Sr reactivity with the YSZ was assessed using dense and porous GDC barriers of various thicknesses fabricated by different techniques such as screen printing, PVD, PLD, and electron beam. The cells were either operated in a potentiostatic/galvanostatic mode or held at an open-circuit voltage (OCV) to separate effects of electrochemical aging from thermal aging. Multiple repeats were used to eliminate the occasional artifacts and atypical trends. In each test, electrochemical characteristics (current and voltage) were measured continuously over time, and the ohmic and polarization resistances were obtained periodically using electrochemical impedance spectroscopy and DRT. Following each test termination, extensive LSCF-GDC post-test characterization was carried out using SEM/EDS and STEM. Advanced machine learning tools were applied to provide the quantitative estimation of the particle/pore sizes and electrode microstructure variation under different operating conditions.
Moore-I1
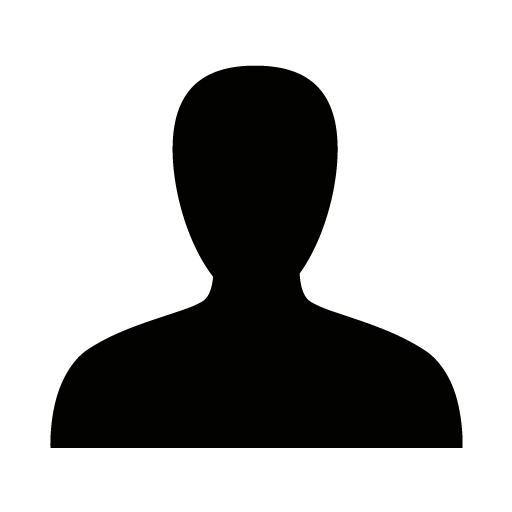
Traditionally, ceramics have been extremely difficult to machine into complex shapes with higher accuracy and efficiency due to their hard and brittle properties. In recent years, laser ablation and 3D-printing are attracting great interest as they offer the possibility of achieving complex shapes [1].
For example, we have recently deal with the mesoscale geometry control of the electrode/electrolyte interfaces for solid oxide cells (SOCs) applications [2]. The idea consists on the fabrication of novel architecture designs of the electrode/electrolyte interfaces at the mesoscale range and compatible with both the microstructure characteristic length (around 1 μm) and the dimensions of the cell (above 100 μm). With these architecture interfaces we intend to increase the current exchange between the different layers and as a consequence, the overall electrical performance of the device. In fact, the electro-chemically active area is proportional to the electrode/electrolyte interface volumetric area, which can be increased by the modification of the interface geometry at the mesoscale characteristic length scale.
Some of the examples will be shown on yttria stabilized zirconia (YSZ) electrolytes. In this sense, a decrease in polarisation with respect to an unprocessed cell was in the range of 30%, tested using symmetrical cells. Similar results were obtained in complete single cells (electrolyte supported), measured in fuel cell mode. Additionally, 3D laser processed surfaces are being also applied on All Solid State Lithium Batteries (ASSBs) electrolytes of LAGP (Li1.3Al0.3Ge1.7(PO4)3) and LLZTO (Li6.4La3Zr1.4Ta0.6O12).
In addition, we will also show the first examples of laser sintering of thin YSZ or CGO (cerium gadolinium oxide) layers, as a novel and rapid sintering technique using fast heating rates and short processing times or low temperatures.
Moore-O1
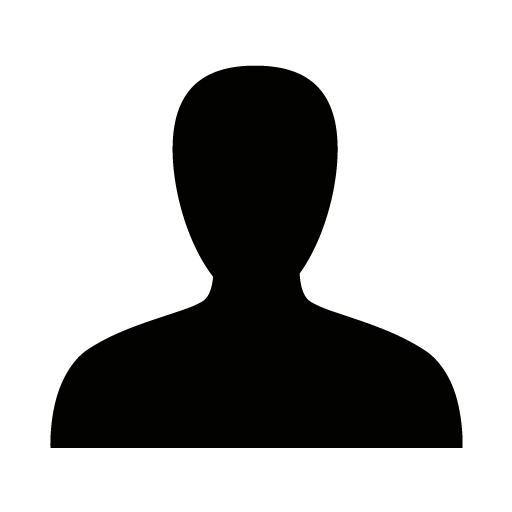
Hydride ions (H−) have attracted attention as fast ionic carriers contributing to the realization of next-generation electrochemical devices. Though several metal hydrides and oxyhydrides with high H− conductivity have been reported,[1-3] the mechanism of fast H− conduction has not yet been fully revealed. The low charge density of H−s leads to large polarizability (αH− = 10.17 Å3),[4] which may provide an inspiration that H−s delocalize and conduct through the sublattice of electropositive cations. This migration feature contrasts with protons with extremely high charge density.
In this work, we report the occurrence of mixed conduction in lanthanum oxyhydrides (LaH3−2xOx) known to be pure H− conductors when hydrogen-blocking electrodes are deposited.[3] We prepared highly sintered LaH3−2xOx pellets with Pd electrodes for hydrogen concentration cells and measured the electro-motive force (emf). The polarity of the emf voltage indicates that the H−s are mobile ion species. However, the ion transference number calculated from the magnitude of emf was not unity but almost 0.5, indicating that H−s and other charge carriers transport in LaH3−2xOx. This talk will discuss identifying charge carriers other than H−s will be discussed based on the results of hydrogen permeation measurements for LaH3−2xOx.
Moore-O2
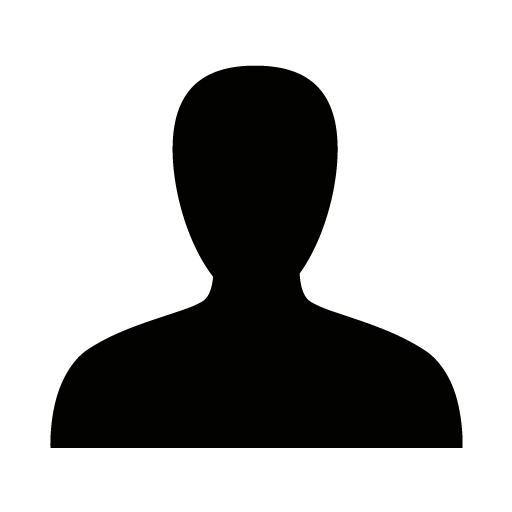
LaH2.75O0.125 is known as an excellent H conductor with an activation barrier of 0.3-0.4 eV. The Arrhenius plot of experimentally measured ionic conductivity versus the reciprocal of temperature (1/T) of LaH2.8O0.1 appears to bend at ~350 K. A transition between two quasi-linear Arrhenius regimes of the self-diffusion coefficient below and above a critical temperature around ~440 K, instead of a single linear Arrhenius regime, cannot be ruled out in using neural network potential (NNP) based molecular dynamics (MD) simulation results of LaH2.75O0.125. [1]
I conducted neural network potential-based molecular dynamics (MD) simulations using the Matlantis package. A critical temperature Tc exists where the activation barrier is 0.35 and 0.20 eV below and above Tc, respectively. Checking the variance of O positions regardless of the time step reveals that O can diffuse when the temperature T>Tc, resulting in low activation barrier paths. Use of MD simulations with various O and La mass points out the coexistence of the original diffusion process with an activation barrier of 0.25 eV and the 0.20 eV process above Tc.
The O and La also have strongly anharmonic characters, which may be contributing to the high ionic conductivity of LaH2.75O0.125.
Moore-O3
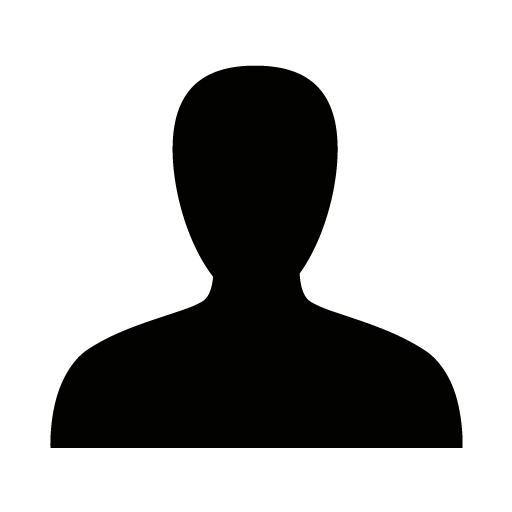
Curriculum Vitae
Jinwook Kim
291, Daehak-ro, Yuseong-gu, Daejeon, Republic of Korea
E-mail: coik8627@kaist.ac.kr
TEL: 82(KOR)-10-9324-8627
RESEARCH INTERESTS
Solid oxide fuel cells, Solid oxide electrolyte cells, Electro-chemical reaction, Thin-film model electrode, Electrode reaction mechanism
RESEARCH SKILLS
- Techniques for fabrication thin film based model electrode (PLD, Sputtering, Photo lithography)
- Techniques for characterization of material properties (IV, EIS, SEM, XRD, XPS, etc.)
- Techniques for fabrication of oxide materials for electrode and electrolyte (Solid-state, Sol-gel synthesis, Uniaxial pressing, etc.)
- Techniques for surface modification (Electrochemical deposition, Infiltration)
- Techniques for synchrotron based in operando surface analysis (XAS, XPS)
EDUCATIONS
2019.03-2023.02, Ph.D., Materials Science and Engineering, KAIST (GPA:4.10/4.30)
(Advisior : Prof. WooChul Jung)
2017.03-2019.02, M.S., Materials Science and Engineering, KAIST (GPA:4.17/4.30)
(Advisior : Prof. WooChul Jung)
2013.03-2017.02, B.S., Materials Science and Engineering, KAIST (GPA:3.83/4.30, Cum Laude)
PUBLICATIONS
1. J. Kim+, G. G. Yang+, W. Lee+. S. Nam, J. Seo, J. K. Kim, S. Kim, H. Kim, Y. Choi, J. W. Han*, B. G. Jeong*, S. O. Kim*, and W. Jung* “Electrochemical Value of Metal Nanocatalysts Supported on Conductive Oxide Electrodes for Oxygen Exchange Reactions” (in preparation)
2. J. Kim+, S. Nam+, H. Kim, S. Ahn, J. H. Kim, B. Jeong*, D. Chen*, and W. Jung* “Protonic Charge Transfer Dominates Hydrogen Incorporation Reaction at Triple Phase Boundary in Proton-Conducting Solid Oxide Cells Fuel Electrode” (in preparation)
3. S. Nam+, J. Kim+, H. Kim, S. Ahn, S. Jeon, Y.Choi*, B.K. Park*, and W.Jung* “Revitalizing oxygen reduction reactivity of composite oxide electrodes via electrochemically deposited PrOx nanocatalysts” (in revision, Advanced Materials)
4. S. Nam+, H. Kim+, J. Kim+, and W.Jung* “Application of Electrochemical Deposition in Solid Oxide Fuel Cell Technology” (in preparation)
5. J. Kim+, H. Kim+, S. Nam, Y.Choi*, and W.Jung* “Exploring gas/solid interface reactions of solid oxide cell electrodes via model thin film systems” (in preparation)
6. Y. Choi+, H. Ha+, J. Kim, H. G. Seo, H. Choi, B. Jeong, J. Yoo, E. Crumlin, G. Henkelman, H. Y. Kim*, and W. Jung* “Unveiling Direct Electrochemical Oxidation of Methane at the Ceria/Gas Interface” (submitted, 2024)
7. H. Shin+, J. Seo+, S. Jeon+, S. J. Jeong, J. Kim, S. Lee and W. Jung* “Enhanced Catalytic Activity and Stability of SOFC Electrodes through Plasma-driven Surface Modification” Journal of Materials Chemistry A (2024)
8. Y.B. Kim+, S. Kim+, J. Kim, J. K. Kim, S. J. Jeong, D. Oh, and W. Jung* “Synthesis of Highly Tunable Alloy Nanocatalyst through Heterogeneous Doping Method” Advanced Science (2022)
9. S. Jeon+, J. Seo+, J.W. Shin+, S. Lee, H.G. Seo, S. Lee, N. Tsvetkov, J. Kim, J. An*, and W. Jung* “Metal-oxide nanocomposite catalyst simultaneously boosts the oxygen reduction reactivity and chemical stability of solid oxide fuel cell cathode” Chemical Engineering Journal (2022)
10. J. Kim+, Y. Choi+, D.-K. Lim, J. Yoo, H. G. Seo, S. Kim, S. Kim, and W. Jung* “Electrochemically plated nickel-decorated ceria nanostructures for direct hydrocarbon solid oxide fuel cell electrodes” Journal of Materials Chemistry A (2022)
11. H. G. Seo, D. H. Kim, J. Seo, S. J. Jeong, J. Kim, H. L. Tuller, J.-W. Son*, and W. Jung* “High-Performance and Durable Fuel Cells using Co/Sr-Free Fluorite-Based Mixed Conducting (Pr,Ce)O2-δ Cathode” Advanced Energy Materials (2022)
12. Y. Choi+, H.-J. Cho+, J. Kim, J.-Y. Kang, J. Seo, J. H. Kim, S. J. Jeong, D.-K. Lim, I.-D. Kim* and W. Jung* “Nanofiber Composites as Highly Active and Robust Anodes for Direct-Hydrocarbon Solid Oxide Fuel Cells” ACS Nano (2022)
13. J. H. Kim, J. Hong, D.-K. Lim, S. Ahn, J. Kim, J. K. Kim, D. Oh, S. Jeon, S.-J. Song*, and W. Jung* “Water as Hole-Predatory Instrument to Create Metal Nanoparticles on Triple-Conducting Oxides” Energy & Environmental Science (2022)
14. J. H. Kim, J. K. Kim, H. G. Seo, D.-K. Lim, S. J. Jeong, J. Seo, J. Kim, and W. Jung* “Ex-Solved Ag Nanocatalysts on a Sr-Free Parent Scaffold Authorize a Highly Efficient Route of Oxygen Reduction” Advanced Functional Materials (2020)
15. Y. Choi, J. Kim, H. G. Seo, H. L. Tuller, and W. Jung* “Nucleation and growth kinetics of electrochemically deposited ceria nanostructures for high-temperature electrocatalysis” Electrochimica Acta (2019)
PATENTS
1. W. Jung, J. Kim, Y. Choi, “METHOD FOR PRODUCING METAL NANOPARTICLES-POROUS OXIDE COMPOSITE STRUCTURE, AND METAL NANOPARTICLES-POROUS OXIDE COMPOSITE STRUCTURE PRODUCED BY THE SAME” (Korea, Registration No. 102173131)
2. W. Jung, S. O. Kim, Y. Choi, S.K. Cha, J. Kim, “Fuel cell electrode and method for preparation thereof” (Korea, Registration No. 102305437)
3. W. Jung, D. G, Lim, J. Kim, “Solid oxide fuel cell comprising anode alkaline-based promoter loaded”
(Korea, Registration No. 102369060 / Japan, Registration No. 7275313 / US, Application No. 17/438,485 / Germany, Application No. 112020001817.2 / China, Application No. 202080023185.1 / Canada, Application No. 3,131,876)
4. W. Jung, J. Kim, D. Oh, “Complex coating layer for Hot Balance of Plant in solid oxide fuel cell”
(Korea, Registration No. 102460522 / Japan, Registration No. 7270775 / US, Application No. 17/604,490)
INTERNATIONAL PRESENTATIONS
1. Solid-State Protonic Conductor-21 (September, 2023, Japan)
Jinwook Kim, Seongwoo Nam, Hyunseung Kim, Sejong Ahn, Woochul Jung* “Investigation of Electrochemical Reactions at the Fuel Electrode Surface in Proton-Conducting Oxide Cells using Metal-Patterned Model Composite Electrodes” (Poster presentation)
2. Petite XII: An International Workshop on the Defect-Chemical Nature of Solids (September, 2023, Germany)
Jinwook Kim, Geon Gug Yang, Sang Ouk Kim, WooChul Jung “Understanding of boosted electrochemical oxygen exchange reaction in nanocatalyst decorated air electrode” (Oral presentation)
3. Solid State Ionics-23 (July 2022, USA)
Jinwook Kim, Geon Gug Yang, Beomgyun Jeong, Yoonseok Choi, Hyunseung Kim, Seongwoo Nam, Jongsu Seo, Jun Kyu Kim, Sang Ouk Kim, WooChul Jung* “Clarifying true value of activated oxygen incorporation reaction by heterogeneous catalyst” (Oral presentation)
4. 44th International Conference and Exposition on Advanced Ceramics and Composites (January 2020, USA)
Jinwook Kim, Yoonseok Choi, JeongDo Yoo, Han Gil Seo, Beomgyun Jeong, Ethan Crumlin, WooChul Jung* “Study of Direct Electrochemical Oxidation of Methane at the Ceria/gas interface” (Oral presentation)
5. Solid State Ionics-22 (June 2019, Korea)
Jinwook Kim, Yoonseok Choi, Jeong Do Yoo, WooChul Jung* “Electrochemical deposition of ceria nanostructure decorated with nickel nanoparticles: A new strategy for improving SOFC electrode performance” (Poster presentation)
AWARDS
1. Researcher of the Year Award, 14th International Symposium of Global Research and Development Centers and Global HUB Centers (November, 2023, Korea)
2. Best Poster Award, Solid-State Protonic Conductor-21 (September, 2023, Japan)
3. Best Poster Award, Samsung Global Technology Symposium 2023 (August, 2023, Korea)
4. Best Poster Award, 2nd Korea SOC symposium (July, 2023, Korea)
5. Best Poster Award, 2021 fall meeting of The Korean Ceramic Society (November, 2021, Korea)
6. Best Poster Award, 2019 spring meeting of The Korean Ceramic Society (April, 2019, Korea)
NATIONAL PROJECTS
National Research Foundation of Korea, “Development of Nanocatalyst Characteristics Evaluation Platform for High Performance Solid Oxied Cell Electrodes”, (RS-2023-00240788), Principal Investigator, 2023-2024 (55000$/Year)
Proton-conducting ceramic cells are emerging as promising alternatives to conventional oxygen ion-conducting devices, offering the potential for low-temperature operation in next-generation energy technologies [1-3]. Despite their promise, a detailed understanding of the electrochemical reactions at the electrodes of proton-conducting ceramic cells remains elusive, impeded by the complex nature of these reactions and a gap in knowledge concerning electrode materials. Notably, the use of model electrodes for in-depth mechanistic studies [4-6]—commonplace in the research of traditional oxygen ion-conducting fuel cells—is scarce for proton-conducting counterparts.
This study focuses on fundamental research of the electrochemical reactions occurring at the fuel electrode surface in proton-conducting cells. Metal patterned BaZr0.4Ce0.4Y0.1Yb0.1O3−δ model composite electrodes are utilized to investigate the electrode reactions. Impedance analysis is conducted under various hydrogen partial pressures and applied voltages to analyze the electrochemical activity. Additionally, in-operando X-ray photoelectron spectroscopy is employed to perform real-time surface chemical analysis under high-temperature with hydrogen exposure conditions and over-potentials. This comprehensive analysis lead to the identification of triple phase boundaries as the dominant active sites and the charge transfer step of protons to metal as the RDS for the hydrogen incorporation reaction among 45 candidate steps. The findings contribute to a comprehensive understanding of the electrochemical processes in proton-conducting ceramic fuel cells, providing valuable insights for the design of fuel electrode materials.
Moore-O4
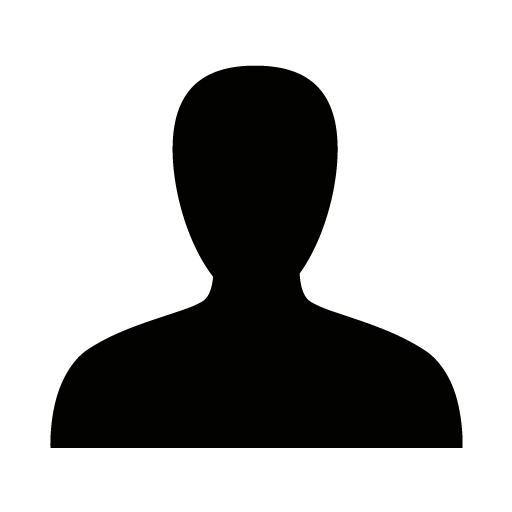
Abstract
Proton conducting ceramic fuel cell (PCFC) is a next generation fuel cell that can operate with high efficiency. One of challenges for the commercialization of PCFC is to reduce the polarization resistance of the cathode [1], and a variety of cathode materials have been investigated. Electrochemical impedance spectroscopy (EIS) with alternating current (AC) is often used to evaluate the polarization resistance of PCFC cathode. In most cases of the analysis, the ionic transport number of the electrolyte is assumed to be 1. However, in typical PCFC electrolytes, the ionic transport number often cannot be assumed as 1 because the electrolyte shows the mixed conduction of proton and electron hole, especially under high temperature and high oxygen partial pressure conditions.[2] Such mixed conduction in the electrolyte may lead to underestimation of the polarization resistance. In this study, using a porous Pt electrode on a typical PCFC electrolyte, BaZr0.8Yb0.2O3-δ (BZYb), as a model case, we aimed to experimentally clarify effect of mixed conduction in the electrolyte on the impedance responses of PCFC cathode. And, we attempted to establish the precise method for evaluating the polarization resistance of PCFC cathode by the fitting with appropriate equivalent circuits considering mixed conduction in the electrolyte.
Experimental
An electrochemical cell was prepared using BaZr0.8Yb0.2O3-δ (BZYb) as a proton conducting electrolyte. On both sides of the electrolyte pellet, porous Pt electrodes were sintered at 1273 K as a working electrode (WE) and a counter electrode (CE). A reference electrode (RE) of porous Pt was set around the side of the electrolyte. The cell was placed in two-chamber configuration, and EIS measurements (AC amplitude: 30 mV, frequency; 106 to 10-3 Hz, under open circuit) were performed with the three-terminal method. Temperature was 773-973 K and the atmospheres were 2% humidified 0.01-100% O2 for the WE, and 2% humidified 5-100% H2 or 0.01-100% O2 for the CE/RE.
Results and discussion
EIS measurements were carried out under various p(O2), while the atmosphere of CE/RE was fixed at wet 5% H2. As results, unique responses were observed. Firstly, the Nyquist plot showed a linear rise in the high frequency region. Secondly, the frequency corresponding to the impedance response was much slower than the general fuel cell cathodic reaction on a Pt electrode. Finally, the x-intercept of the Nyquist plot, which is generally interpreted as the ohmic resistance of the electrolyte, shifted toward higher resistance side as p(O2) decreased. It was considered that these responses were caused by the change in electronic hole conduction associated with the change in oxygen nonstoichiometry of the electrolyte. In fact, the calculated capacitance [3] assuming the oxygen nonstoichiometry change of the electrolyte due to the electrode polarization was in good agreement with the observed capacitance. In order to confirm effect of hole conduction to the impedance response, EIS measurements were performed under the constant WE atmosphere of wet 100% O2, while varying the CE/RE atmosphere from wet 100% H2 to wet 100% O2. The impedance response decreased drastically when the CE/RE atmosphere changed from H2 to O2, even though the WE atmosphere was constant. This indicated that EIS measurements tend to underestimate the polarization resistance of PCFC cathode because of the influence of minor electronic conduction in the electrolyte, and, in most cases, the estimation of the polarization resistance by the spectrum fitting assuming a conventional simple R//C circuit is not appropriate.
Abbey-K1
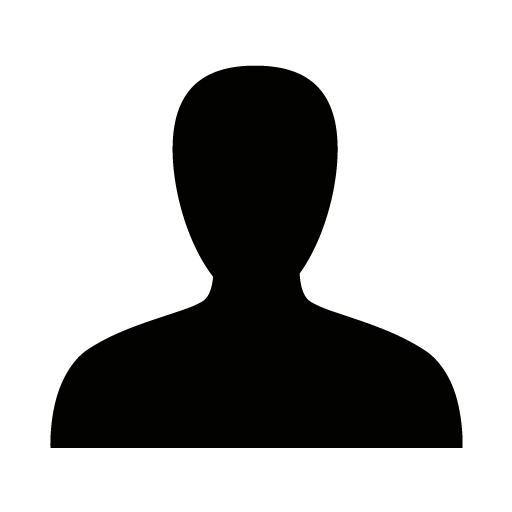
The sustainability of the process industry, transportation, and energy management will rely on low-CO2 technologies and renewable electricity. The volatile nature of renewable energy sources requires new energy-storage tools together with novel, highly efficient methods to electrify unitary steps in the industry. Here, we present a microwave-driven redox activation of solid-state ionic-conducting materials. Ceramic oxides, such as doped CeO2 and ZrO2, can be chemically reduced at unprecedented low temperatures (<220 °C) by the sole application of microwave radiation, leading to an instantaneous outstanding rise in electrical conductivity. Reproducible, reversible and cyclable surface release of O2 is dependent on material, microwave power, and radiation. Evaluation of different trivalent dopants in CeO2 reveals that microwave-driven reduction correlates with lattice and electronic properties. Narrower oxide bandgaps allow for higher electronic conductivity requiring lower temperatures to trigger the microwave-driven reduction, while larger ionic lattice sizes enhance oxygen diffusion and release. The ability of microwave radiation to evolve O2 and transmute the redox catalytic behaviour in oxides can be used in the electrification of several catalytic processes, such as the partial oxidation of methane to produce olefins or syngas, and as a new tool for the formation of catalytic nanoparticles via exsolution. Direct formation of molecular energy carriers, such as H2 and CO, is possible through further reaction of the redox-activated solid material and low-energy molecules via a deoxygenation mechanism.
Abbey-I1
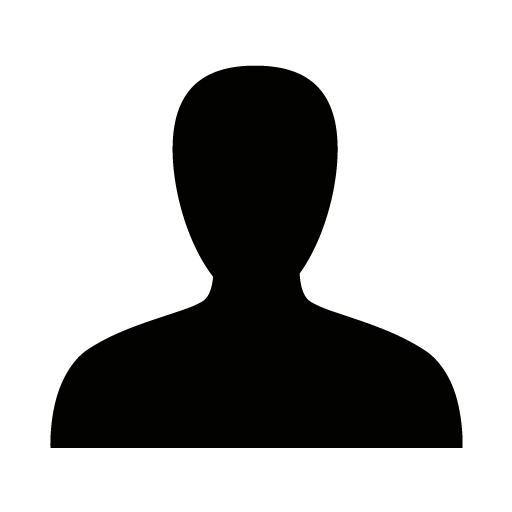
HYDROGENi (https://hydrogeni.no/) is a Centre for Environment-friendly Energy Research (FME), which seeks to develop expertise and promote innovation through focus on long-term research in selected areas of environment-friendly energy. The HYDROGENi centre aims to build a sustainable hydrogen economy with focus on four main research areas: Cost-efficient and scalable production of hydrogen and ammonia; transport and storage in Norway and Europe; end-use technologies; safety and material integrity. HYDROGENi’s activities are a collaborative effort from over 50 Norwegian and European partners from both research and industry covering the entire H2 value chain. In addition, HYDROGENi has the largest ever academic research programme in an FME and aims to educate 35 PhD/postdoc students and over 100 MSc/BSc candidates.
The presentation will give a brief overview of the Centre's activities promoting a technology-neutral approach to clean H2 and NH3 production and scaling-up of production capacity needed for domestic use and export. Several electrolyser technologies are integrated in the centre and represented by technology developers and users: Proton exchange polymer based electrolysis, PEMEL, alkaline electrolysis, AEL, anion exchange membrane electrolysis, AEMEL and proton conducting ceramic electrolysis, PCCEL. The work on these technologies encompasses research on materials (primarily catalysts and electrodes) and manufacturing of cells for optimum performance and durability, study of efficiency and dynamic behaviour of electrolysers through a combination of modelling and experimental testing using dedicated test protocols, and design, operation and process integration of large-scale electrolysers in various applications. In this presentation, we will focus on the work carried out on PCCEL technology, presenting results achieved on materials research and multi-scale multi-physics modelling bridging atomistic scale, electrode, electrolyte and cell.
Abbey-O1
The surface area is a critical region for determining oxygen reduction reactions (ORRs) on the cathode in the design of advanced proton-conducting solid oxide fuel cells (H-SOFCs) systems. The particle surface of Sr-containing perovskite is fully covered with Sr segregation, as confirmed by low-energy ionic scattering (LEIS). The Sr segregation layer exhibits poor electronic and ionic conductivity, thereby obstructing ionic conductivity between particles. To enhance surface performance, impregnation was employed to promote catalysis and conductivity at a low cost. Specifically, (001)-oriented Co3O4 was carefully selected and prepared due to its excellent ORR catalysis performance, while (001) was chosen for its lattice constant match. Transmission electron microscopy (TEM) analysis confirmed that Co3O4 adheres well to La0.5Sr0.5FeO3 (LSF), and TEM-energy dispersive x-ray spectroscopy (EDS) revealed inter-diffusion of Fe and Co with a smaller band gap and a strong ability for oxygen vacancy formation, as supported by density functional theory (DFT) calculations. A significant improvement in power density was observed in a single-cell test.
Abbey-O2
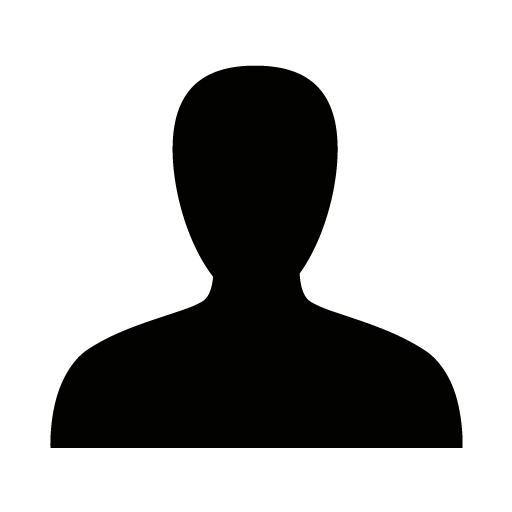
When Max Born looked back to his life's work, he concluded: "I have restricted my work to ideal crystals though I am aware that the theory of the defects in real crystals is practically far more important. This I have left to a younger generation." Indeed, ionic point defects play an important role in determining the phase, structure, properties and functionality of oxide ceramics and thin films. In this talk, I am going to show the methodology and case studies towards a better understanding of the role of ionic point defects (e.g., oxygen vacancies and protons) in determining the activity of oxide electrocatalysts. I will start with our recent work on well-controlled model thin film electrocatalysts for the high-temperature oxygen incorporation reaction (OIR). Combining operando X-ray photoemission spectroscopy and electrochemical measurements, we reveal the change of reaction mechanisms across the phase boundaries triggered by the change of oxygen vacancy concentration. Then I am going to show the encountered complexity when we switch from well-controlled high-temperature solid/gas interfaces to room-temperature solid/liquid interfaces. I will show our recent effort of understanding the room-temperature defect chemistry in model electrocatalysts for the oxygen evolution reaction (OER). Lastly, I will compare the two cases, i.e., high-temperature solid/gas interfaces and room-temperature solid/liquid interfaces, and discuss the similarities and differences towards a better mechanistic understanding of OIR/OER reactions.
Abbey-O3
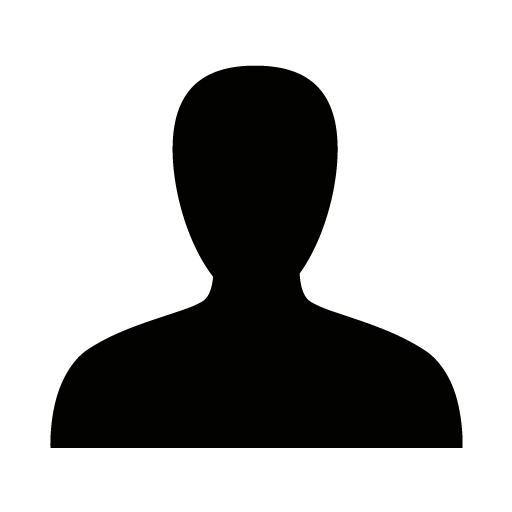
Solid Oxide Fuel Cells (SOFCs) are among the most promising technologies for the production of electricity and heat from traditional and renewable energy sources. In the event of low electricity consumption, excess electrical energy can also be stored in hydrogen produced in the electrolysis mode of SOFCs. However, excellent power outputs of SOFCs are recorded rather at a high temperature range (around 800 °C). The high operational temperature of SOFCs significantly contributes to the high operational costs, and limits the choice of device materials, rendering SOFCs commercially unviable. To enhance economic competitiveness, it is imperative to lower the operational temperature to a range of ≤600 °C while still maintaining a high-power output. Therefore, the development of new electrodes with enhanced electrocatalytic properties is indispensable for boosting the performance of SOFCs. The design of dual-phase electrodes bringing a synergistic effect, significantly benefits the performance of SOFCs, including the fabrication of heterostructured electrodes by mechanically milling or self-assembling techniques [1], in situ exsolution of secondary phase in the form of nanocatalysts [2]. Electrodes with unique dual-phase synergy substantially boost the electrochemical performance of SOFCs [1-4].
In this study, various approaches to fabricate hybrid electrodes have been employed and discussed, aiming to significantly enhance the performance of SOFCs.Sr2Fe2-xWxO6-δ perovskite electrodes with in situ exsolved metallic nanoparticles were designed. Excellent power output was recorded for constructed symmetrical solid oxide cells based on selected hybrid electrodes, showing 679 mW cm-2 at 800°C in H2 and 451 mW cm-2 in wet CH4. The self-assembling technique was employed for the manufacturing nanofiber-structured electrodes with GdBa0.5Sr0.5CoCuO5+δ-Ce0.9Gd0.1O1.95 dual phases, extensively promoting the electrochemical performance of hybrid electrode at intermediate temperature range (≤700 °C). In addition, we have utilized the mechanical milling method to fabricate high performance triple conducting composite with La0.6Sr0.4Co0.2Fe0.8O3–δ-BaCe0.9–xMoxY0.1O3–δ boosting the electrochemical performance of oxygen electrodes for SOFCs, with an excellent power density of 418.7 mW cm-2 at 600°C over a 500 h period.
Gielgud-K1
Avner Rothschild is a Professor of Materials Science and Engineering and the Deputy of the Senior Vice President for Sustainability at the Technion – Israel Institute of Technology. He studied physics and materials engineering at the Technion, and graduated in 2003 with a PhD on thin film metal-oxide gas sensors. After a three-year postdoc on solid-state ionics at MIT he returned to the Technion as a faculty member at the Department of Materials Science and Engineering, and the head of the Electrochemical Materials & Devices research group. His research focuses on electrochemical and photoelectrochemical materials and devices for water splitting as a means of sustainable production of green hydrogen. Professor Rothschild is a co-founder of H2Pro, a startup company that develops a breakthrough water splitting technology for low-cost production of green hydrogen at scale. He was a member of several European consortia and had an ERC consolidator grant on photoelectrochemical water splitting. He is a Fellow of the Royal Society of Chemistry and a Kavli fellow of the National Academy of Sciences USA. Professor Rothschild has received distinguished prizes and awards, including the Samson Prime Minister's Prize for Global Innovation in Alternative Fuels (2020), the Royal Society of Chemistry’s Horizon Prize (2022), and the Climate Solutions Prize for Israel Breakthrough Research (2022).
Water electrolysis presents challenges to increase performance, economic value, and deployment. These challenges result from the co-production of hydrogen and oxygen in the same electrolytic cell, as well as from substantial energy losses. To prevent hydrogen and oxygen mixing, the electrolytic cell is divided by a membrane into cathodic and anodic compartments. This membrane architecture complicates the electrolyzers construction by adding gaskets and compression plates to prevent gas crossover, increasing cost and weight, and limiting high pressure operation. In addition, substantial energy losses, mostly due to the difficult oxygen evolution reaction (OER), increase the cost of energy.
To overcome these challenges, we developed alternative processes that decouple the generation of hydrogen and oxygen into: (1) separate electrolytic cells with alternating auxiliary buffer electrodes that mediate the ion exchange between the anodic and cathodic reactions [1]; (2) consecutive electrochemical and thermally-activated chemical stages in the same cell [2]; and (3) separate electrolytic and catalytic cells [3]. These configurations avoid the need for expensive membranes and sealing, leading to membraneless electrolysis architectures. In addition, by dividing the OER, a difficult electrochemical reaction that requires four electrons and protons to generate an O2 molecule on a single atomic reaction site, into electrochemical and chemical sub-reactions that occur on four sites [2] or in soluble redox couple [3], the energy loss for oxygen evolution is reduced, leading to high efficiency. The advantages and challenges of the different approaches will be discussed at the conference.
Gielgud-I1
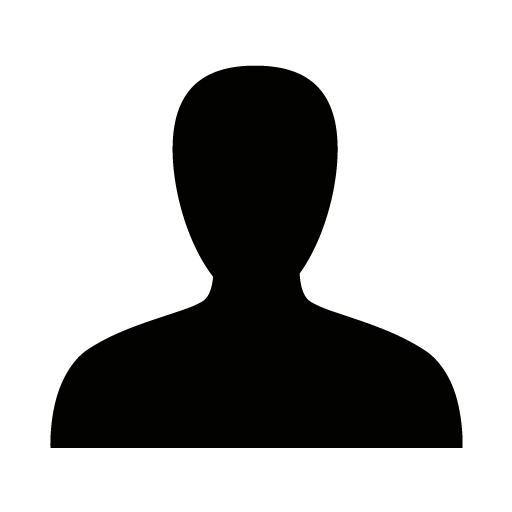
Biogas is an important renewable energy resource that can be effectively converted into electrical energy using solid oxide fuel cells (SOFCs). Traditional Ni-YSZ cermet anodes are not suitable for operation with fuels such as natural gas or biogas due to fast degradation caused by coking and poisoning by ppm levels of H2S, a naturally occurring fuel impurity [1,2]. Perovskite-like oxides are considered a promising alternative to nickel with enhanced tolerance to carbon deposition and sulfur tolerance, although each candidate material has its advantages and drawbacks [1-3]. The aim of the present work is the evaluation of AVO3- and AMoO3-based oxides (A = Ca, Sr) as prospective anode components with an emphasis on the properties relevant for the anode applications.
Selected perovskite oxides including Sr(La)V(Nb)O3-δ, CaVO3-δ, PrVO3-δ and Sr(Mo,V,Ti)O3-δ were prepared under reducing conditions and assessed by a variety of techniques including measurements of electrical properties as a function of T-p(O2), determination of phase stability limits, electrical and dimensional changes on redox cycling, and behavior in presence of H2S and CH4. A(V,Mo)O3-δ-based components exhibit high electrical conductivity under fuel electrode operation conditions, up to 1000 S/cm. The phase stability domain of the perovskite phase is limited but can be extended by donor-type substitutions. While A(V,Mo)O3-δ have poor tolerance against H2S, substituted Sr(La)V(Nb)O3-δ and Sr(Mo,V,Ti)O3-δ perovskites as well as PrVO3-δ show a good stability in the presence of hydrogen sulfide. The introduction of a minor A-site deficiency has no positive effect on the phase stability limits or tolerance towards sulfur contamination. An example of composite electrode material prepared by in-situ reduction of oxidized precursors is demonstrated.
Gielgud-O1
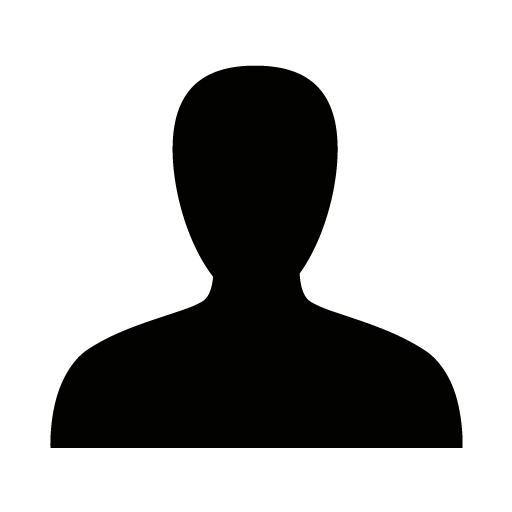
Ternary oxides AmBnOx are used in numerous devices that are exposed to various thermodynamic forces, such as oxygen chemical potential gradient (e.g. solid oxide fuel cells), electric potential gradient (e.g. multi-layered ceramic capacitors), temperature gradient (e.g. thermoelectrics), stress gradient (e.g. piezoelectrics), etc. The materials range from purely ionic conductors to mixed conductors and semiconductors. When these ternary oxides are exposed to external driving forces, especially at high temperatures where the ions that compose the oxide are mobile, the ions migrate, in general with different mobilities. The resulting phenomena of kinetic unmixing and kinetic decomposition have been investigated for many years. However, in most cases severe approximations have been used, such as treating the ternary oxide as a quasi-binary system, and most often only the steady state was considered. Here we analyze the kinetics of unmixing phenomena using a full defect-chemical description of the oxides under consideration. First, we will discuss the kinetics of the Hebb-Wagner polarization kinetics and the implications for bulk resistive switching using SrTiO3 as a model system [1,2]. Second we analyze the kinetics of unmixing of all mobile defects in a ternary oxide using BaTiO3 as a model system. In this way, we can predict the lifetime of the oxide until kinetic decomposition [3].
Gielgud-O2
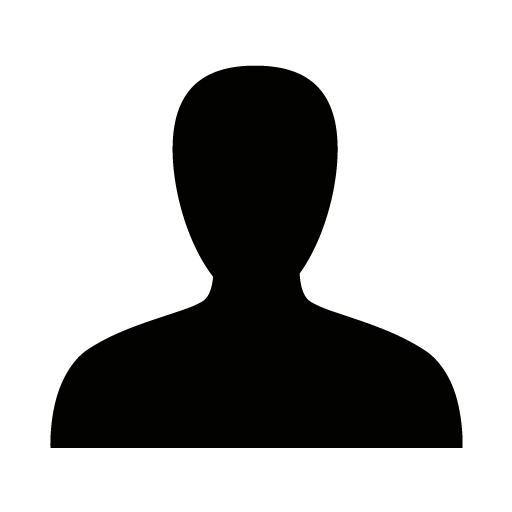
Oxygen exchange kinetics is a critical parameter for the improvement and understanding of fuel cells and solid oxide electrolysers. To enhance those kinetics, electrode materials require good ionic and electronic transport properties. However, other parameters can also influence these kinetics, such as the redox properties of the transition metal that make up the electrode material. Indeed, transition metals are directly involved in the charge transfer reaction that turns gaseous O2 into lattice O2-. However, studying of redox properties is not straightforward, because attempts at modifying the redox properties, for example by doping, will lead to a change in the transport properties. Therefore, it is difficult to separate the influence of redox and transport properties on oxygen reaction kinetics.
This work proposes a new approach to study redox effects during oxygen reaction with an electrode without changing the transport properties of the electrode material. First, a mixed conducting oxide is selected to provide charge transport (here Pr0.1Ce0.9O2-δ)[1], while a transition metal oxide with a spinel type structure is infiltrated at its surface to control the redox properties of the system. Then, the oxygen exchange kinetics of the composite is measured by electrical conductivity relaxation. By varying the spinel composition, it is possible to modify the redox properties without changing the transport properties of the system. The method is applied to the MgAl2-xFexO4 spinel system, in which only Fe is redox active, while Mg and Al act as indirect force to modify the redox properties of the Fe centres while maintaining the spinel structure. Variations of Oxygen exchange kinetics are discussed as a function of composition, site distribution, and oxidation states of the iron in the spinel infiltrate[2] [3]. The analysis of site distribution and oxidation states is performed via Electron Energy Loss Spectroscopy (EELS) and X-ray diffraction.
Gielgud-O3
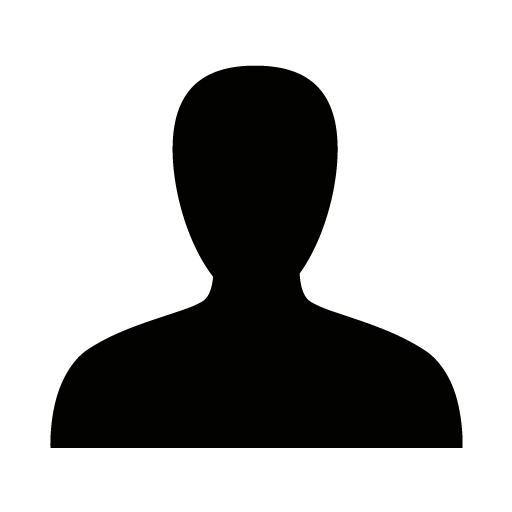
Solid oxide electrochemical cells (SOCs) are among the most promising eco-friendly energy conversion devices, showcasing superior power generation and green hydrogen production abilities. The current primary objective in the field of SOCs research is to lower the operating temperature to ensure thermal cycling stability and minimize system costs. Achieving feasible performance for commercial usage at reduced temperatures necessitates the use of state-of-the-art cobalt-based oxygen electrode materials with ceria/zirconia bilayer electrolytes. Another approach involves increasing electrochemical active sites by constructing nano-sized electrodes. Nevertheless, the fabrication of dense ceria/zirconia bilayers and nano-structured electrodes remains challenging due to inevitable chemical reactions and the intricate sintering nature of materials.
In this study, we simultaneously tuned the microstructures of electrolytes and electrodes via an ultra-fast one-step microwave-assisted sintering method, successfully fabricating dense and defect-free ceria/zirconia bilayers and nano-structured Ni-zirconia fuel electrodes. The sintering process was conducted at a relatively low temperature of 1200°C and took only several tens of times less than the conventional method. This effectively mitigated undesirable chemical reactions between different phases and suppressed the coarsening of fuel electrode components. The developed SOCs displayed exceptional performance in both the fuel cell and electrolysis modes. Furthermore, we investigated the relationship between electrochemical performance and structural features using a digital twinning approach combined with 3D-reconstruction techniques.
Gielgud-O4
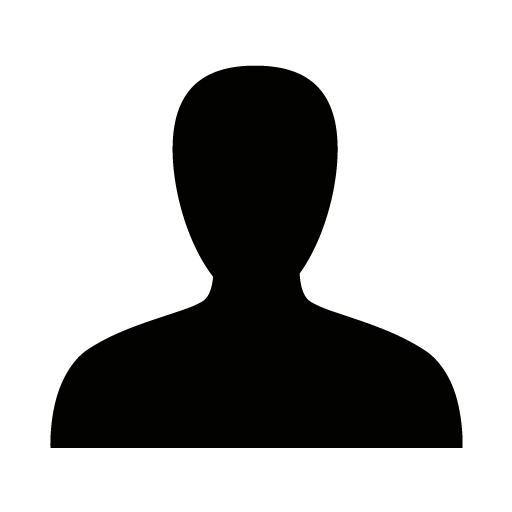
The current collector is a crucial component in Solid Oxide Fuel Cells (SOFC) and Solid Oxide Electrolysis Cells (SOEC) stacks, serving to transfer the electricity generated within the cells through interconnects. Its role significantly influences the overall performance of the stack. CuMn oxide, utilized for the current collector, possesses a spinel structure known for its robust electrical conductivity and exceptional durability, attributes that stem from its stable structural composition.To optimize the performance of the current collector, we engaged in a comprehensive selection process for the Cu:Mn composition ratio. This involved analyzing various sets of Area Specific Resistance (ASR) data, each corresponding to different composition ratios of CuMn oxides. Subsequently, we chose the optimal Cu:Mn composition ratio based on the analysis and proceeded to manufacture CuMn foam with this specific ratio.Upon the manufacturing of CuMn foam, we conducted thorough observations and assessments of the ASR data associated with the foam possessing the optimal composition ratio. The results were promising, indicating favorable performance in terms of electrical conductivity and durability. Encouraged by these findings, we moved forward to implement the CuMn foam as a current collector within the SOFC and SOEC stacks. In doing so, we integrated the CuMn foam current collector into the stacks and rigorously evaluated the overall performance of the stacks.
Fleming-I1
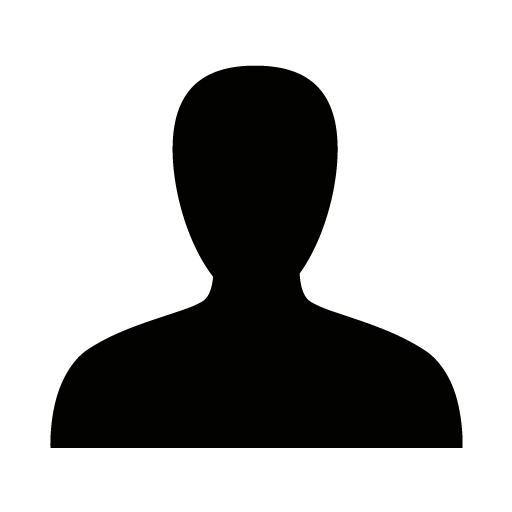
Atmospheric Pressure Spatial Atomic Layer Deposition (AP-SALD) is a recent variant of ALD that offers fast processing while preserving the unique assets of ALD, namely, precise thickness control down to the nanometer, high-quality films even at low temperatures, and unique conformality. As a result, AP-SALD is ideal for applications requiring high throughput at low cost, such as new generation energy devices. In particular, the AP-SALD approach based on close-proximity deposition heads is highly versatile since it can be easily customized by proper design of the deposition heads and because the deposition takes place in the open air without the need of any deposition chamber.
But there is more to AP-SALD than a faster and scalable version of ALD. In particular, In my talk I will illustrate how 3D printing can be used to prototype and customize close-proximity deposition heads, and how in so doing SALD can indeed be tuned to deposit custom patterns without the need of pre-patterning steps, or be adapted to tubular substrates such as separation membranes. I will then present our studies showing the effect of open-air processing on the properties of the thin films deposited with our close-proximity system, and how the choice of precursor can have a huge impact on the final properties of the materials deposited. I will finally present recent results on the deposition of functional thin films for electrochemical energy devices.
Fleming-O1
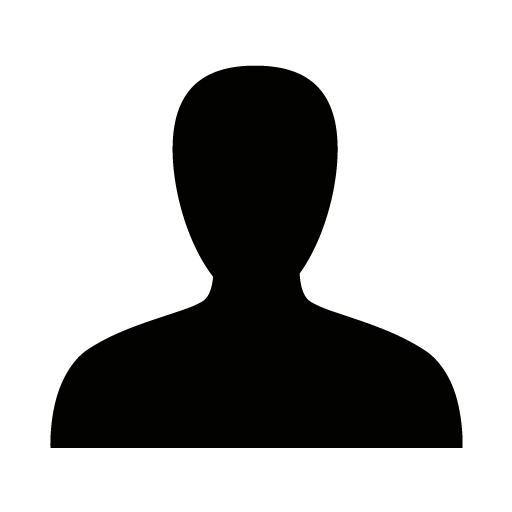
Lithium-ion batteries have garnered attention due to their high energy density, extended lifespan, and efficiency 1. However, safety issues associated with their organic liquid electrolyte 2, which can react exothermically in accidents or reduce lifespan under extreme conditions, have prompted exploration into alternatives. The substitution of liquid electrolytes with solid-state electrolytes is considered due to their enhanced thermal stability, potentially extending battery life and increasing capacity 3,4. Despite these advantages, challenges such as interfacial resistance, interface reactions, and low ionic conductivity 5,6 persist, along with the need for synthesis methods that allow precise control over material morphology, structure, composition, and industrial scalability. In this context, sol-gel processing stands out as a versatile option for such applications.
Among various families of solid electrolytes, perovskite-type ones (ABO3, where A= Li and La; B= Ti) have gained significance due to their environmental stability and diverse applications. These electrolytes exhibit grain ionic conductivities exceeding 10⁻³ S/cm 7–9. However, the total conductivity remains in the order of 10⁻³ due to high ionic conductivity at grain boundaries 10–12.
The study focuses on synthesizing the solid electrolyte Li0.3La0.57TiO3 (LLTO) using the sol-gel method with nitrates, alkoxides, and complexing agents. The research explores the impact of different calcination temperatures on composition, structure, morphology, and ionic conductivity, evaluating their influence on reducing grain boundary resistance.
Results reveal that the sol-gel process produces solid electrolytes with a perovskite structure and phase percentages exceeding 91% in powders calcined at temperatures of 700, 800, and 900 °C, surpassing 99% in pellets sintered at 1300 °C. These exhibit a tetragonal crystalline system with the p4/mmm space group. Powders calcined at various temperatures present spherical particles ranging from 50 to 100 nm, with a uniform composition. After sintering at 1300 °C, pellets achieve a relative density exceeding 95%.
Calcination temperature significantly influences electrochemical properties. At 900 °C, grain boundary ionic conductivity reaches 0.34 mS/cm, with a total ionic conductivity of 0.3 mS/cm at 30 °C. Overall, solid electrolytes calcined at temperatures above 800 °C exhibit grain boundary conductivity exceeding 0.10 mS/cm, attributed to proper crystallization, increased density, reduced porosity, and a higher concentration of vacancies, facilitating rapid ionic diffusion of lithium in the solid electrolyte. Consequently, materials obtained through this sol-gel process demonstrate significant potential for use in fully solid-state lithium-ion batteries.
Fleming-O2
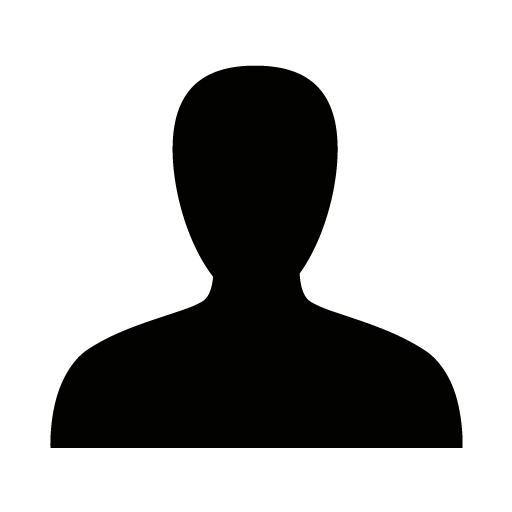
In developing all-solid-state batteries with oxide-based materials, the densification of ceramic electrolytes (CE) is a key step to allow circulation of lithium ions. Traditional sintering techniques require high temperatures that often lead to unwanted interactions between the CE and active materials, undermining battery performance.
This research presents an alternative approach using the glass-forming abilities of certain CEs that can facilitate sintering at lower temperatures. When glass is heated above its glass transition temperature, it transforms into a viscous phase that allows for simultaneous densification and crystallization. If the glass transition temperature and crystallization temperature are lower than the sintering temperature, the resulting thermal treatment leads to a more conductive and denser CE compared to an already crystallized one subjected to the same treatment. We use a Nasicon-type ceramic electrolyte—specifically, Li1.5Al0.5Ge1.5(PO4)3 (LAGP)—to explore this method.
The study investigates the crystallization behavior of LAGP and its impact on ionic conductivity. Our results demonstrate an improvement in the density and conductive properties of the glass-ceramic electrolyte with a significatively lower temperature treatment. Moreover, X-ray diffraction techniques are employed to monitor the progression of crystallinity, revealing that an autocatalytic nucleation process allows for earlier lithium-ion pathway creation.
This finding provides meaningful insights into the production of ASSBs that could avoid the drawbacks of high-temperature assembly.
Westminster-I1
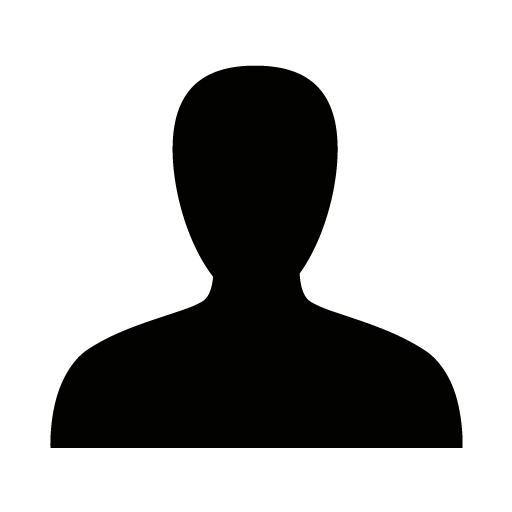
Recently, message-passing graph neural network interatomic potentials (GNN-IPs), particularly those with equivariant representations such as NequIP, have attracted significant attention due to their data efficiency and high accuracy. Moreover, the atomic embedding vector in GNN-IPs allows for training over large datasets with diverse chemistry, resulting in pretrained, general-purpose machine learning force fields. In this presentation, we introduce the development of an efficient parallelization scheme compatible with GNN-IPs and its implementation into a package named SevenNet (Scalable EquiVariance-Enabled Neural NETwork), which is based on the NequIP architecture. Through benchmark tests on a 32-GPU cluster with examples of SiO2, SevenNet achieves over 80% parallel efficiency in weak-scaling scenarios and exhibits nearly ideal strong-scaling performance as long as GPUs are fully utilized. We then pre-train SevenNet with a vast dataset from the Materials Project (dubbed ‘SevenNet-0’) and demonstrate its out-of-distribution generalization capabilities. We subsequently apply SevenNet-0 to investigate electrolytes in lithium batteries, such as screening organic molecules in liquid electrolytes and identifying new solid-state electrolytes.
Westminster-O1
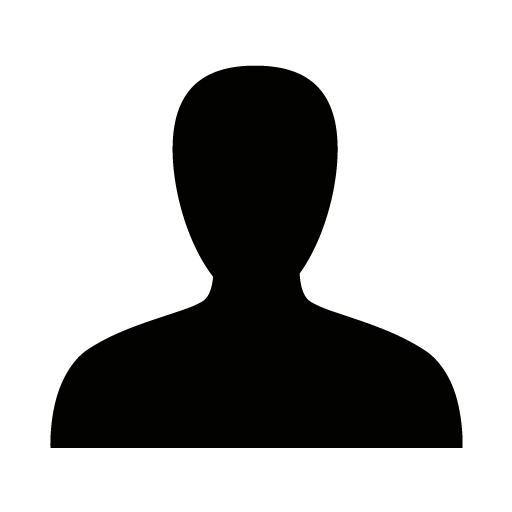
In its high-temperature cubic δ-phase, pure bismuth oxide demonstrates the highest oxide ion conductivity among all solids, reaching approximately 1 S cm-1 just above 1000 K. However, a phase transition occurs below this temperature, causing a sudden decline in conductivity. Substantial scientific efforts have been directed towards developing a bismuth oxide-based compound that maintains the high conductivity of the δ-phase at lower temperatures. Various approaches, such as doping or creating materials where bismuth is not the predominant cation, have been explored. In this study, we illustrate how a comprehensive understanding of conduction processes in new solid electrolytes can be attained by combining experimental methods with theoretical modeling, employing a molecular dynamics approach
We utilize diverse Density Functional Theory (DFT) methods, primarily employing versions of the general gradient approximation in molecular dynamics, especially at elevated temperatures reaching up to 1200 K. Depending on the specific issue under investigation, we adjust the conventional ab initio approach by incorporating van der Waals interactions or Hubbard corrections as necessary. All simulations are performed using Vienna Ab initio Simulation Package. Calculations are typically conducted on systems ranging from as much as three hundred atoms, especially when modeling lighter species, to as few as one hundred atoms for compounds predominantly composed of heavy ions.
In the perovskite sodium bismuth titanate, Bi0.5Na0.5TiO3, we elucidate the modulation of ionic conductivity through the composition-dependent existence of polarons, resulting in the peculiar formation of clusters of oxide ion vacancies. This phenomenon has been employed to account for the observed sudden decrease in ionic conductivity when subtle adjustments are made to the Bi/Na ratio, as observed in experiments. In a similar pervoskite system, 0.2(Ba0.4Sr0.6TiO3)-0.8(Bi0.5Na0.5TiO3), the integration of high-resolution powder neutron diffraction, impedance spectroscopy, and ab initio calculations unveils that titanium makes a contribution to the overall polarization that is less than one-third in magnitude. Notably, the displacements of oxygen ions and A-site cations, especially bismuth, play a crucial role. In the case of the rhombohedral form of praseodymium doped Bi2O3 we show how the experimentally observed phase transition of the anion sublattice can be explained by the rotation of BiO3 quasi-molecule, driven by the presence of the 6s2 electron lone pair of the bismuth cation.
Westminster-O2
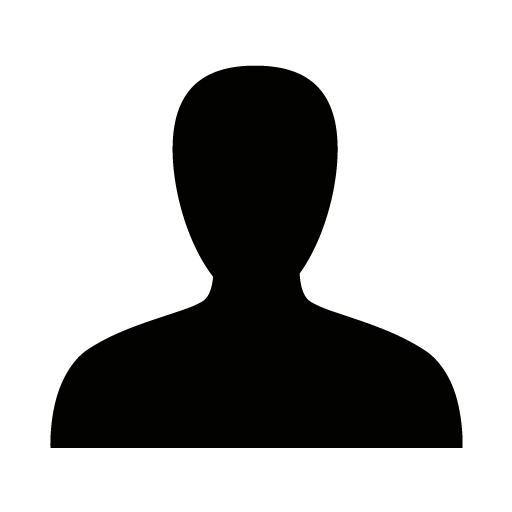
The ever-growing demand for electrochemical energy storage has brought great attention to solid-state battery research in recent years, with solid-state sodium batteries being already commercially available today. In parallel, it has become evident, that thermal management is an indispensable factor for optimizing battery lifetime and performance. The NASICON-substitution series (Na1+xZr2P3-xSixO12, x=0-3) is one of the most well studied and promising sodium solid-electrolytes as they combine high stability with reasonably good ionic conductivity. However, deep knowledge of thermal transport behavior in this and other solid electrolytes is often lacking, and many connections remain elusive.
In this work the thermal conductivities of Na1Zr2P3O12 and Na4Zr2Si3O12 are assessed experimentally and by ab-initio modelling in a wide temperature range of 2 K to 773 K. Anharmonic lattice dynamics calculations are combined with a 2-channel model to demonstrate that both, phonon-gas like and heat transport via local random walk of heat (so-called diffusons) are present in NASICON compounds. It is intuitively shown how phonon linewidth broadening leads to a saturating behavior of thermal conductivity opposed to the 1/T decay found for the classic phonon-gas model. Additionally, detailed evaluations of the phonon density of states by projection on atoms, sites, and in jump direction are joined with temperature dependent X-ray diffraction to provide insights how the jumps of sodium ions are driven by their vibrational spectrum. This presentation will display how lattice dynamics calculations as well as experimental methods can be used to obtain a more holistic picture of ionic and thermal transport in the NASICON-series and unravel their interplay.
Westminster-O3
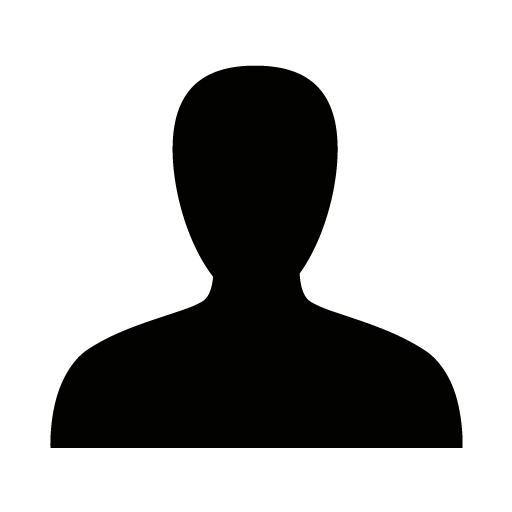
Electroconductive metal−organic frameworks (MOFs) have emerged as high-performance electrode materials for supercapacitors, but the fundamental understanding of the underlying chemical processes is limited. Here, the electrochemical interface of Cu3(HHTP)2 (HHTP =2,3,6,7,10,11-hexahydroxytriphenylene) with an organic electrolyte is investigated using a multiscale quantum-mechanics/molecular-mechanics (QM/MM) procedure and experimental electrochemical measurements. Our simulations reproduce the observed capacitance values and reveals the polarization phenomena of the nano porous framework. We find that excess charges mainly form on the organic ligand, and cation dominated charging mechanisms give rise to greater capacitance. The spatially confined electric double-layer structure is further manipulated by changing the ligand from HHTP to HITP (HITP= 2,3,6,7,10,11-hexaiminotriphenylene). This minimal change to the electrode framework not only increases the capacitance but also increases the self-diffusion coefficients of in-pore electrolytes. The performance of MOF-based supercapacitors can be systematically controlled by modifying the ligating group.
St.James-O1
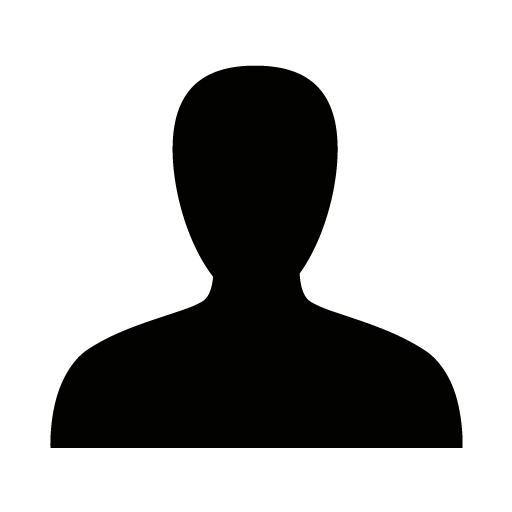
The Li10GeP2S12 are promising candidates of solid electrolytes for all-solid-state batteries, because it shows the high lithium ionic conductivity of 12 mS cm-1 at room temperature. For practical use, Li10GeP2S12 should be synthesized by the liquid-phase method, which enables mass production. However, Li10GeP2S12 synthesized by this solution synthesis shows lower ionic conductivity than that of the mechanical milling [1]. In this study, Li10GeP2S12 were synthesized through the solution synthesis with the Ti boat at the heat treatment step as the improved version. In addition, their particle characteristics of the sample synthesized by the solution synthesis were analyzed by transmission electron microscopy, particle size analysis, the impedance measurements at low temperatures at 190 K, and X-ray photoelectron spectroscopy. The solution synthesis sample in this study exhibited an ionic conductivity of 5.5 mS cm-1, which is the highest ionic conductivity of previous studies of liquid phase synthesis. The solution synthesis sample exhibited the smaller particle size than that of the mechanical milling sample. This smaller particle size at the solution synthesis sample is correlate with the higher grain boundary resistance, which cause to show lower total ionic conductivity. Moreover, the surface layer from solvent was detected on the particle surface synthesized by the solution synthesis based on the XPS measurement. This surface layer contributes to show the higher stable interface of Li-In/Li10GeP2S12. We expect these findings to enable the effective harnessing of the particle states to develop sulfide solid electrolytes with higher ionic conductivity and fine particles.
St.James-O2
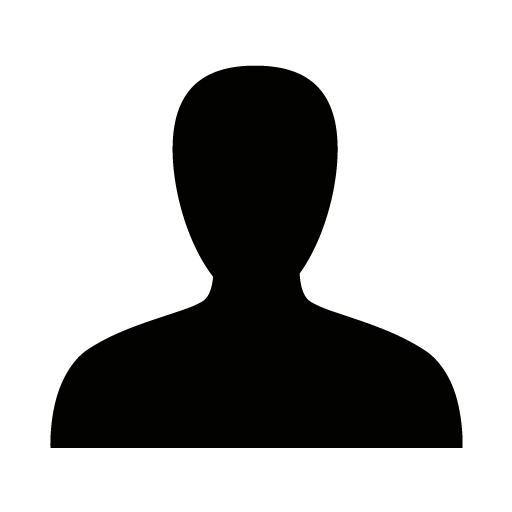
All-solid state batteries are promising candidates to achieve high energy densities and enable the use of high capacity anodes such as lithium metal or silicon alloys. Over the last years, intensive efforts have been made to improve the ionic conductivity of solid electrolytes and to gain detailed knowledge of the structure and transport behavior in their pristine state. However, for the preparation of cathode composites, processing methods as ball milling are applied to mix the ionic conductors with cathode active materials. While these post-synthesis processing steps are applied very frequently in the preparation of solid-state batteries, little is known about how exactly the processing step influences the properties of the solid electrolyte.
Here, we use an extensive set of experimental methods to fundamentally study the effects of post-synthesis ball milling on the highly conducting chloride-rich argyrodite Li5.5PS4.5Cl1.5. Investigating the crystallinity by X-ray diffraction and neutron pair distribution function shows that ball milling clearly decreases the crystallite size of the ionic conductor. In combination with nuclear magnetic resonance spectroscopy, we observed that the general local structure is maintained with a slight increase of disorder. The reduced crystallite size correlates with a decrease in ionic conductivity in the processed solid electrolytes. Nevertheless, the smaller particles lead to lower tortuosity when fabricating cathode composites, overall affecting performance. Optimization of these processing conditions can play a significant role on the road to highly performing solid state batteries.
St.James-O3
Prof Neelam Srivastava did her Graduation and post-graduation from Gorakhpur University and PhD from Banaras Hindu University. Dr. Neelam Srivastava (Professor of Physics, BHU, Varanasi) has been working in the field of solid state ionics since 1989, with special emphasis on polymer electrolytes synthesis and characterization. Her group is presently dedicated to synthesize economical, environment friendly, high and fast ion conducting polymer-in-salt polymer electrolyte membranes for all-solid-state flexible electrochemical devices. The group is presently exploring starches from different botanical origins to achieve the target and optimize the starch-salt combination and its composition for commercial application. A special synthesis protocol developed by the group resulted in low cost, easy to synthesize, free standing transparent flexible electrolyte membrane, and is being successfully tested for fabrication of electrochemical devices such as all-solid-state-flexible-supercapacitor, microbial fuel cell etc. The group has already submitted three patents (one granted, one approved waiting for NBA certificate and third under examination). Besides the material synthesis and characterization her group is also involved in understanding the ion transport mechanism in polymer electrolytes. The group has developed a methodology for impedance data analysis under conductivity formalism to extract the information regarding the applicability of different format of conductivity power law equations. She has delivered talks in many international and national conferences/ workshop/ refresher courses etc. and organized national and international conferences in the field of material sciences and energy devices. Prof Srivastava has many national and international collaborations. Prof Srivastava's group has handled 04 projects and has 35 international publications and 05 book chapters to their credit. Prof Srivastava has organized many national and international conferences. She is specially dedicated for the popularization of science at school level, for which she is working under the NASI Varanasi chapter and has organized school students visits to BHU UG and research labs. Prof. Neelam Srivastava has received the INSA visiting scientist fellowship in 2014. In year 2022 BHU recognized her as “Wonder Woman” and under the aegis of NASI-Varanasi Chapter and GATI-BHU, Rector Banaras Hindu University has facilitated her for scientific achievements.
Economical, Ecofriendly and Easy to Handle Polymer-In-Salt-Electrolytes
Neelam Srivastava, Dipti Yadav, Kanak Aggarwal
Department of Physics (MMV Section), Banaras Hindu University, Varanasi-221005, India
neel@bhu.ac.in
Polymer-In-Salt-Electrolytes (PISEs) [1] have attracted scientific community since 1990s when the Angell’s [2] group has indicated that if the salt concentration is increased beyond the threshold value so that the ion-clusters are connected throughout the matrix, then the ion dynamics changes. At this concentration ion transport is decoupled from polymer segmental motion and now ions diffuse through ion-cluster and hence it is much faster. It is also reported that at high salt concentration the cationic transference number also enhances. Hence depending upon the salt concentration the polymer electrolytes are classified into two classes i) Salt-In-Polymer-Electrolytes (SIPEs) - salt concentration is lower than the polymer and ii) Polymer-In-Salt-Electrolytes (PISEs)- salt concentration is higher than the polymer. SIPEs, which are presently used at commercial level, needs to be improved on two main fronts i) slow ion movement and ii) poor cationic transference numbers and hence PISEs are highly desired materials. Unfortunately, suitable polymer host which can hold a large amount of salt and keep it in dissociated form is still to be recognized, to take PISEs to commercial level. When it comes to commercial application, the cost, ease of synthesis/ handling and ecofriendly nature are also equally important.
Literature reports that for PISEs synthesis it is important to have the salt/ salt-mixture in molten state so that when polymer is added the rubbery state may remain intact. Hence as per reported protocols one has to identify suitable salt-mixture and then suitable host. Still reported polymer-salt combinations suffers recrystallization of salt, brittleness of morphology and also aging affects resulting in poor electrochemical and/or mechanical properties making it unsuitable for commercial application. It is found that PISEs reported needs further thermal treatments/additions of additives to control the recrystallization and aging effects.
Our group [3-4] has developed a simple solution casting protocol for synthesis of an economical, ecofriendly and easy to handle PISEs from crosslinked starches, where there is no need of getting the molten state salt/salt-mixture. The thought process behind this protocol and selection of starch/ crosslinked starch as host polymer is that the salt breaks the starch into smaller molecules resulting in generation new –OH and –H to interact with salt, i.e. increasing salt concentration itself creates a favorable atmosphere for its acceptance. Starch is hydrophilic in nature and presence of large amount of salt adds up to it, and such materials have moisture content varying from ~5% to 25%, depending to salt and starch combination, which is a favorable property leading the synthesized PISEs to behave as Water-In-Polymer-Salt-Electrolytes (WiPSEs) [5]. By exposing the freshly synthesized samples to high humidity these materials were stabilized with respect to ambient humidity changes. These materials lead to ESR <10Ω (reaching to as low as <1Ω) and wide electrochemical stability window (ESW>2.5V) and ion relaxation time below µSec. The supercapacitor fabricated, using synthesized PISEs with commonly available supercapacitor electrodes, have the behavior at par to other electrolytes reported in literature. With lab synthesized activated carbons synthesized in laboratory, the capacity up to 150F/g has been obtained with columbic efficiency >98%. Since the synthesis protocol and chemical used are economical, the starch based PISEs are economical and also environment benign (because starch is renewable polymer). The material is flexible and can be molded in desired shape and size and hence is a potential candidate to reach at commercial level.
St.James-O4
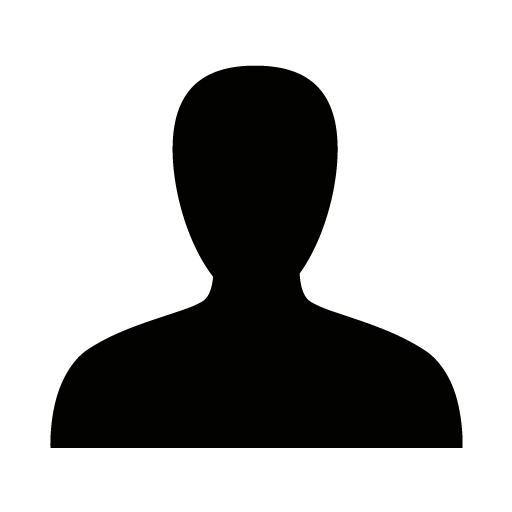
The influence of microstructure on ionic conductivity and cell performance is a topic of broad scientific interest in solid-state batteries. The current understanding is that interfacial decomposition reactions during cycling induce local strain at the interfaces between solid electrolytes and anode/cathode, as well as within the electrode composites. Investigating the effects of internal strain on ion transport is particularly important given the significant local chemomechanical effects caused by volumetric changes of the active materials during cycling. Here, we characterize the induced strain in Li6PS5Br structure under applied pressure up to 10 GPa, with in-situ high pressure synchrotron diffraction and ex-situ powder diffraction. A permanent strain is observed in the material even after pressure release, indicating long-range strain fields typical for dislocations. Pair distribution function analysis reveals no change in the short-range local structure, but smaller coherence length for the more strained material is found, indicating that the origin of the long-range strain is caused by dislocations. With increasing strain, dislocation densities are also found. With increasing strain, an increase in the lithium ionic conductivity can be observed that extends into an improved ionic transport in solid-state battery electrode composites. This work shows the potential of strain engineering as an additional approach for tuning ion conductors in solid-state batteries without changing the composition of the material itself.
Moore-I1
Solid Oxide Fuel Cells are renowned for their unique fuel flexibility. Even state of the art anode supported cells, exhibiting an anode functional layer and a substrate based on Ni/8YSZ-cermets, can convert a wide variety of fuels as reformed hydrocarbons, carbon monoxide, ammonia, ethanol and AdBlueTM besides hydrogen [1]. In case of ceria based fuel electrodes the stability towards contamination can be even improved [2].
In this contribution performance of SOFCs operated with different fuels respectively reformates thereof will be discussed. A particular focus will be on stability issues and the observed interactions of fuel electrode and substrate with these fuels and hardly avoidable contaminants as sulfur species [3,4] and higher hydrocarbon residues acting as soot precursors [5]. Examples of these interactions will be illustrated by means of cell performance degradation, changes in the impedance spectrum and distribution of relaxation times [6] as well as microstructural changes and macroscale defects observed in post-test analyses. Possible countermeasures by the expensive adjustment of the operating conditions on the system level or modifications of the fuel electrode itself will be discussed.
Moore-O1
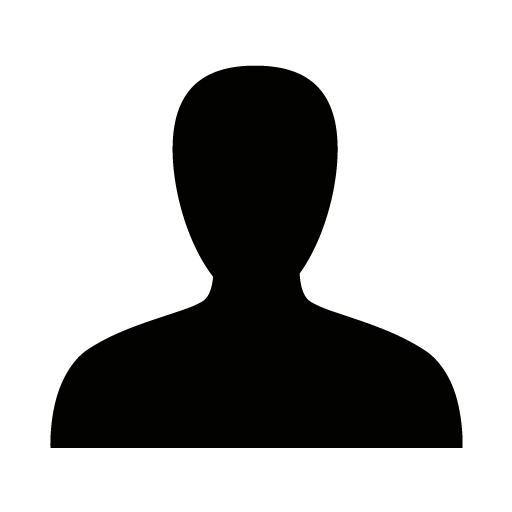
Reversible protonic ceramic electrochemical cells (R-PCECs) offer a promising avenue for energy conversion and storage at low to intermediate temperatures (400-700°C), facilitating the conversion of water to hydrogen in electrolysis mode and vice versa, generating electricity in fuel cell mode [1-2]. However, challenges such as slow oxygen reduction/evolution reaction (ORR/OER) kinetics at these temperatures, along with undesirable surface segregation and structural deterioration, hinder their development. PrBaCo2O5+δ (PBC) has emerged as a focus for oxygen electrode materials due to its notable catalytic activity and electrical conductivity. Nonetheless, issues like cobalt oxides and barium carbonate segregation at the surface/interface impede oxygen ion and proton diffusion, leading to a swift decline in performance [3]. This presentation outlines our strategies to enhance the surface/interface of PBC using doping, infiltration [3], and chemical etching techniques. Applied with precision, these methods create a surface/interface that is both more active and durable, thus improving oxygen electrode functionality. Employing advanced characterization tools like Transmission Electron Microscopy (TEM) and Electron Energy Loss Spectroscopy (EELS), we have achieved a comprehensive understanding of the microstructural and compositional transformations from the bulk material to the surface/interface, enabling significant advancements in R-PCECs technology.
Moore-O2
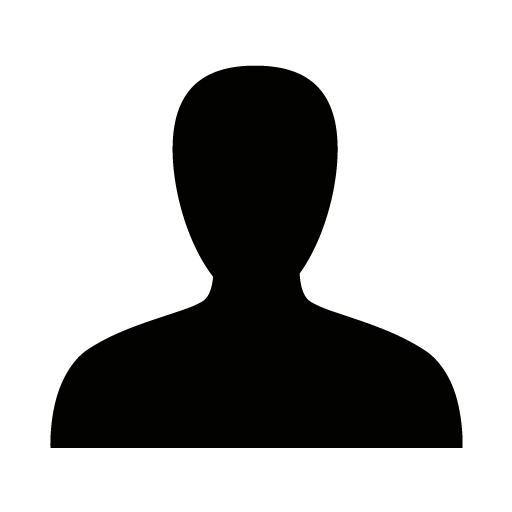
Triple ionic-electronic conducting oxides, in which electron holes, oxygen ions, and protons are simultaneously mobile, are garnering massive attention as potential oxygen electrodes in protonic ceramic cells. While their high electrocatalytic activities and proton transport abilities generally provide opportunities for enhanced performance of the electrochemical cells, the lack of fundamental knowledge on the proton uptake mechanism hinders the rational modification and design of the materials. Herein, we investigate the proton uptake mechanism in double-perovskite PrBa0.5Sr0.5Co1.5Fe0.5O5+δ (PBSCF), a candidate material exhibiting the triple ionic-electronic conducting property. The proton solubility of PBSCF is examined by direct observation of proton using secondary ion mass spectrometry. We then comprehensively discuss the correlations between previously hypothesized proton uptake mechanisms (hydration vs hydrogenation) and experimentally measured properties such as mass and conductivity changes. This work provides a guideline for establishing the design principles of triple ionic-electronic conducting oxides based on mechanistic understanding.
Moore-O3
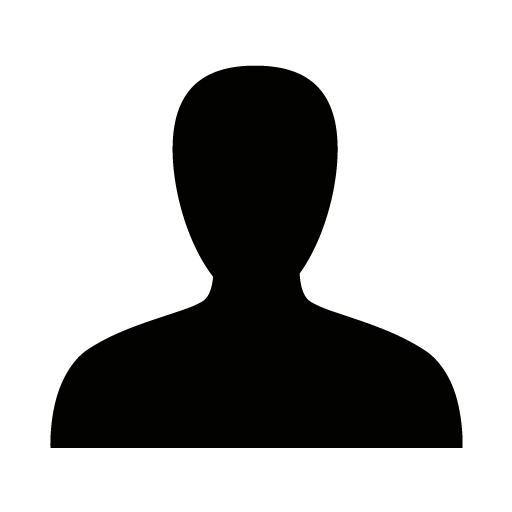
Micro- and nano-electronic devices based on metal-oxide-metal (MOM) and complementary metal-oxide-semiconductor (CMOS) systems are the basis of modern technology. Over many decades, scaling down of transistor- and memory-device dimensions have led to exponential advances in computing power. This scaling has also led to the replacement of SiO2 with higher dielectric constant materials, such as HfO2. However, in spite of decades of perfection, oxides used in CMOS devices, including SiO2 and HfO2, are prone to many field-induced reliability issues[1]. Here, we present the results of multi-scale modelling which link the physical processes responsible for the field induced degradation of amorphous (a-) HfO2 with the characteristics of time-dependent dielectric breakdown (TDDB), such as temporal evolution of current through the oxide and Weibull plots of TDDB. Model parameters characterizing the creation of oxygen vacancies and the electron tunneling process are calculated using Density Functional Theory (DFT), employing a hybrid density functional. The vacancy-generation model is based on previous work, which shows that electrons trap into deep, intrinsic states in a-HfO2[2] . The trapping of electrons into these states facilitates the production of vacancies by lowering the activation energy for vacancy-interstitial pair generation[3]. It is found that subsequent trapping of electrons at vacancies also facilitates the production of additional vacancies. We calculate a range of parameters associated with these defects and reactions and use this information to parameterize a device level simulation in the Ginestra code[4]. Effects such as TAT, Fowler-Nordheim tunneling, heat generation and vacancy generation et cetera are all included. This allows us to simulate the time evolution current through a TiN/HfO2/TiN subjected to electrical stress, accounting for how stress-induced carrier injection and vacancy-interstitial pair generation are inter-related and ultimately affect the oxide properties. The results show that the trap based model agrees well with experiments on the field-induced degradation of HfO2. We also gain an insight into the formation of the percolation pathway from the spatial distribution of generated vacancies. This also confirms the Joule feedback mechanism, in which so-called hard breakdown is caused by local heating around a percolation pathway, leading to a catastrophic increase and current and generation of vacancies.
Abbey-I1
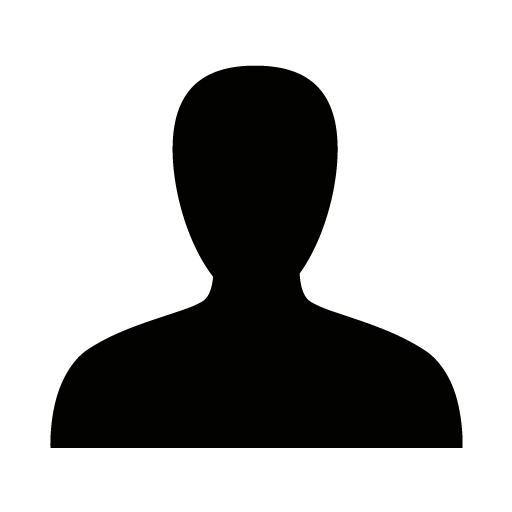
Understanding functional materials for energy conversion or storage devices requires profound knowledge of their surface as well as their bulk properties. The bulk can often be sufficiently described by defect formation and trapping and equilibrium concentrations for electronic as well as ionic carriers. The surface, in particular at operating conditions, is much stronger influenced by additional factors such as impurities, interactions with the gas phase or near-surface restructuring effects. In further contrast to the bulk, local charge neutrality doesn’t have to be maintained. This enables dipole formation with extremely strong implications on the concentration of charged defects, charge transfer processes and the kinetics of oxygen exchange reactions. The importance of understanding these phenomena is highlighted by the large scatter of experimentally determined oxygen surface exchange parameters in literature of common MIECs, that have the same bulk composition but different surfaces, see ToC Graphic.
In this contribution, recent experimental results of in-situ impedance spectroscopy during pulsed laser deposition (i-PLD) will be presented that show the excellent performance of several MIEC oxygen electrodes for solid oxide cells in the pristine state and their degradation of surface exchange kinetics upon exposure to acidic gases. Similar adverse effects could also be achieved by using acidic oxide overlayers of 0.05-0.5 nm nominal thickness. Most importantly, also improving the surface kinetics is possible for most common MIEC materials by depositing decoration layers of basic oxides. Despite the consistent direction of changes (basic surface = higher activity and vice versa) that was observed for several materials, the exact mechanistic effect on either activation energy, prefactor or specific defect concentrations is often unclear and will be discussed.
In addition to indirect evidence on dipole formation observable in electrochemical measurements, we also found direct evidence for dipoles from both electrons as well as ions of a MIEC. Electronic evidence are substantial changes in the work function created by sub-monolayer decoration layers. Ionic evidence was found in ToF-SIMS measurements that due to the sampling depth of 1-2 nm allowed simultaneous detection of the respective decoration layer and the host MIEC. Considerable changes in secondary ion formation due to surface-near dipole formation were observed and are discussed together with site-specific properties of decoration ions as well as the stability of a sub-nm decoration layer towards diffusion into the MIEC.
Abbey-O1
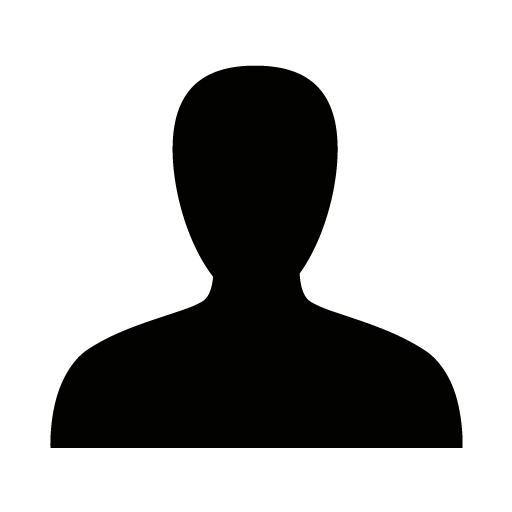
Solid Oxide Cells (SOC) offer an effective and eco-friendly method for hydrogen generation or conversion, with a potential for their integration with renewable energy sources for energy storage and distribution. SOC operating in electrolysis mode are also notable for their capability to produce higher added-value chemicals, such as oxygen, carbon monoxide, syngas, ammonia, or light hydrocarbons by means of the so-called Power to X routes.
Conventional SOC devices use catalytically active Ni-based electrodes, which imposes stringent requirements on the fabrication and operation conditions. In particular, Ni-containing electrodes must be maintained in strongly reducing atmospheres at the fuel side, both during the cell operation or in stand-by mode, in order to prevent nickel oxidation and associated mechanical issues. The concept of symmetric cells (sSOC) proposes utilization of the same electrode material at both oxygen and fuel side, simplifying the procedure of the cell fabrication and improving device tolerance to switching between gases supplied to either electrode. Redox-stable Sr2Fe1.5Mo0.5O6-δ (SFM) with a notable catalytic activity is considered as a promising electrode material for sSOCs, whereas its application does not require using hydrogen at the fuel electrode side, increasing the safety of the device.
The present study focuses on electrochemical characterization of SFM and SFM-GDC composite as electrodes for electrolyte-supported sSOCs. The cell configuration included dense membranes of 6Yb4ScSZ electrolyte with remarkable ionic conductivity, protective layers of GDC, coated with electrode layers. SFM and SFM-GDC electrodes have a pronounced activity towards steam electrolysis, with a strong influence of water supply to the fuel electrode on the performance. Particularly, small-area SFM/6Yb4ScSZ/SFM symmetric button cells deliver the electrolysis currents up to 1-1.4 A/cm2 at a cell voltage of 1.3 V and can operate upon periodic switches between the fuel and air atmospheres. The electrodes are active towards co-electrolysis of H2O-CO2 mixture, with somewhat lower activity with respect to CO2 conversion in comparison with conventional Ni-based composites.
Among the factors affecting the performance and stability of the electrochemical cell, one could highlight the phase stability of the electrode material, mechanical and microstructural features of the electrode layers, especially in hydrogen- and water-containing atmospheres. The cell characteristics are shown to be strongly susceptible towards high-temperature interactions between the components, which necessitates a proper optimization of the fabrication procedure. Deposition of SFM-GDC functional layers improves the mechanical stability of the electrodes, without compromising the electrochemical properties. Optimized procedures of the electrode deposition are currently being implemented for fabrication of large-area (up to 40 cm2) single-repeating units with SFM and SFM-GDC symmetric electrodes.
Abbey-O2
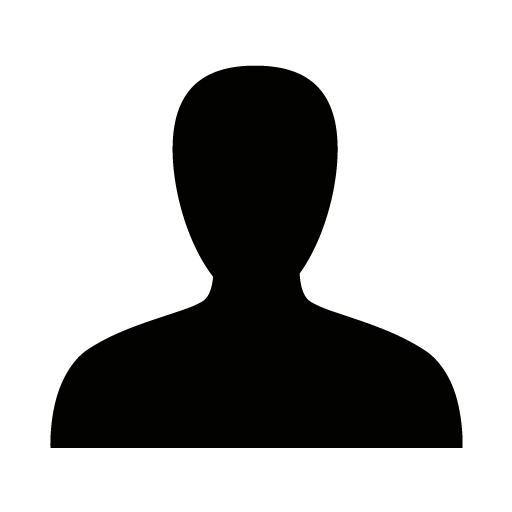
Solid oxide fuel cells (SOFC) are a promising energy conversion and storage technology that can be one of the crucial elements of sustainable development policy, significantly contributing to the increasing share of clean energy. During the oversupply of energy from renewable sources, hydrogen (fuel) can be produced in the electrolysis mode of SOFCs, which could be later consumed to produce electricity and heat as needed in fuel cell mode. Typically, the operating temperature of SOFCs is higher than 800 °C and this raises issues primarily with thermal compatibility and material degradation, which significantly affects costs and makes SOFCs uneconomical. At the desired operating temperature below 600 °C, most electrode materials lose their excellent electrochemical properties and the performance of SOFCs decreases radically. Therefore, the fabrication of new highly-efficient electrodes operating at lower temperatures is essential for the development of economical and high-performance SOFCs. Recently, heterostructured electrode materials obtained by various techniques, including mechanically milling, infiltration, in situ assembling and in situ exsolution of nanoparticles, have become increasingly popular for the development of highly-efficient electrodes. The undoubted advantage of fabricating heterostructured electrodes includes the high mixed ionic-electronic conductivity (MIEC) and enhanced catalytic activities [1-3].
In this work, new high-performance heterostructured electrodes consisting of double perovskite GdBa0.5Sr0.5CoCuO5+δ (GBSCC) and Ce0.9Gd0.1O1.95 (GDC) were fabricated by different strategies, including: mechanical milling, one-pot synthesis, self-assembling nanofibrous electrodes by electrospinning. The synergistic effect of engineering heterostructured electrodes with different content ratios of GBSCC and GDC was systematically investigated. It was found that the self-assembling nanofibrous heterostructured electrodes present the most excellent electrochemical performance (0.05 Ω cm2 at 700 °C), and a stable performance was recorded for more than 500 hours. The cathode polarization and oxygen transport mechanism of heterostructured electrodes were investigated in the function of oxygen partial pressure and by the distribution of relaxation times (DRT) method. High-performance SOFCs were constructed with the 70%GBSCC+30%GDC heterostructured electrode, showing an excellent power output of 1023 mWcm-2 at 725 °C. In addition, heterostructured electrodes via the formation of A-site diffident LaxSr2-xFe1.4Ti0.2M0.2Ni0.1Co0.1O6-δ (La = Sm, Pr; M = Mo, W, Cr, Mn) perovskites with in situ exsolved nanoparticles were designed. The in situ exsolution of nanoparticles on the surface of nanofibrous electrode was investigated for boosting the performance of SOFCs.
Abbey-O3
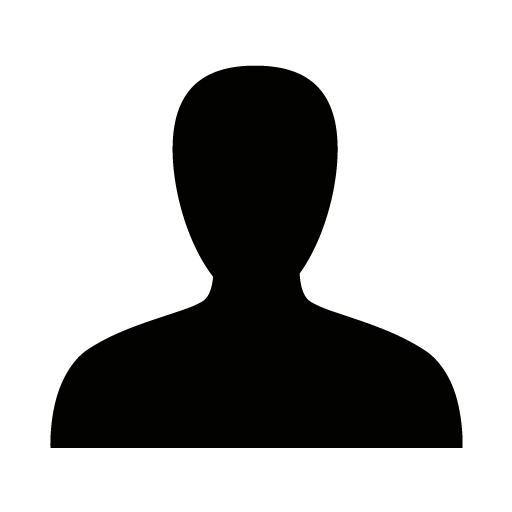
Mr. Wu is currently a Phd student at the University of St Andrews, School of Chemistry. He was born in Hebei, China and obtained his Bachelor' s Degree in Electrical and Electronic Engineering from University of Electronic Science and Technology of China in 2022. In the same year, he also achieved a second Bachelor' s Degree in Engineering from University of Glasgow. During his undergraduate years, he furthered his education as a visiting student at Westlake University and gained practical experience as a technologist at Ningbo Keningda Industry Company Limited. His previous research projects primarily centered on the use of hydrogen pumps in the treatment of nuclear wastewater. Haodong joined in JTSI group and started his PhD studies on Oct 2022. His research will undertake the structural optimization method for high temperature CO2 utilization.
In response to energy shortages, the imperative to develop clean energy sources is paramount for fostering the sustainable advancement of contemporary society. Given the persisting challenges in hydrogen storage and transportation, solid oxide fuel cells (SOFCs) fueled by hydrocarbons have emerged as a focal point of scientific investigation. However, the performance degradation caused by carbon deposition, resulting from incomplete hydrocarbon oxidation on conventional nickel-based cermet anodes in SOFCs, significantly impairs its potential for widespread commercial viability. The Zr0.1Ce0.9O2 catalyst has demonstrated outstanding anti-carbon deposition capability coupled with elevated oxide-ion conductivity. Nevertheless, the electrochemical performance of the ceramic electrode is subpar in comparison to that achieved with a Ni-cermet catalyst.
Herein, we report a carbon-resistant anode with Zr0.1Ce0.85X0.05O2-δ-SrFe0.75Mo0.25O3-δ (X = Fe, Co, Ni, Cu) (ZDC_X-SFM) composite structure. ZDC_X nanoparticles were synthesized using the Sol-gel method. Notably, strained Fe, Co, Ni and Cu nanoparticles can be synthesized on the ZDC bulk through hydrogen annealing, with their surface morphology is modifiable by adjusting the doping concentration and annealing temperature. Significantly, compared to other samples, the introduction of Cu significantly enhanced grain growth. Characterization using X-ray diffraction (XRD), X-ray photoelectron spectroscopy (XPS) and Raman spectroscopy indicated alterations in lattice strain within the catalyst, accompanied by a concomitant rise in surface oxygen vacancy concentration during metal exsolution. The doping of ZDC with Fe, Ni, and Cu leads to a notable increase in its cubic lattice parameters, which escalate from 5.3876 Å to 5.3881 Å for Fe, 5.3889 Å for Ni, and 5.3887 Å for Cu. Only cobalt doping led to a slight reduction in lattice parameters, decreasing from 5.3876 Å to 5.3875 Å. Doping with different transition metals consistently resulted in an increased ratio of Ce3+.
After being mixed with SFM, the electronic conductivity of ZDC_X increased, rendering it suitable as an anode material. A single cell was fabricated with ZDC_X-SFM as the anode, La0.8Sr0.2Ga0.8Mg0.2O3−δ (LSGM) as the electrolyte, and La0.6Sr0.4Co0.2Fe0.8O3-δ (LSCF) as the cathode. Numerous electrochemical characterizations were performed to evaluate its performance. Electrochemical impedance spectroscopy analysis reveals a reduction in polarization resistance. Additionally, the primary reaction process and the rate-limiting step were further clarified through the analysis of relaxation time distribution. Overall, this study demonstrates a stable and active composite catalyst for energy applications.
Gielgud-K1
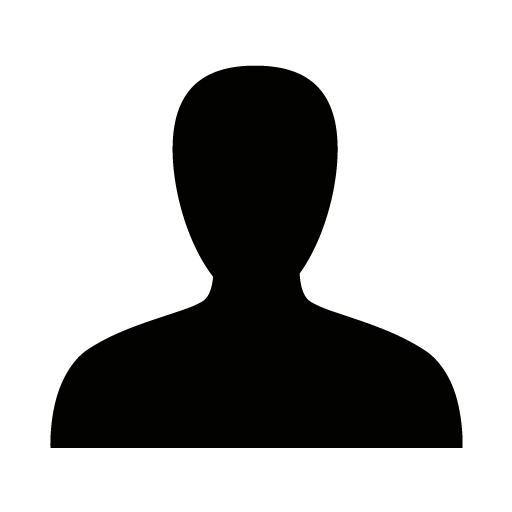
Proton and oxide-ion conductors are attractive materials having a wide range of potential applications such as PCFC, PCEC, SOFC, and SOEC. The conventional strategy to improve the proton and oxide-ion conductivity is acceptor doping into oxides without oxygen vacancies. However, the acceptor doping results in proton and vacancy/oxide-ion trapping near dopants, leading to the high apparent activation energy and low proton conductivity at intermediate and low temperatures. Intrinsic oxygen vacancies are the oxygen vacancies in a parent material. In this keynote, I present the high proton and oxide-ion conduction via the intrinsic oxygen vacancies.
The hypothetical cubic perovskite BaScO2.5 may have intrinsic oxygen vacancies without the acceptor doping. Herein, I report that the cubic perovskite-type BaSc0.8Mo0.2O2.8 stabilized by Mo donor-doing into BaScO2.5 exhibits high proton conductivity within the ‘Norby gap’ (e.g., 0.01 S cm−1 at 320 °C) and extremely high chemical stability under oxidizing, reducing and CO2 atmospheres [1]. The high proton conductivity of BaSc0.8Mo0.2O2.8 at intermediate and low temperatures is attributable to high proton concentration, high proton mobility due to reduced proton trapping, and three-dimensional proton diffusion in the cubic perovskite stabilized by the Mo-doping into BaScO2.5. The donor doping into the perovskite with disordered intrinsic oxygen vacancies would be a viable strategy towards high proton conductivity at intermediate and low temperatures. I also report high proton and oxide-ion conduction in hexagonal perovskite-related oxides with intrinsically deficient oxygen layers [2-7].
Many Bi-containing compounds exhibit high oxide-ion conductivity via conventional vacancy mechanism. However, interstitial oxide-ion conduction is rare in Bi-containing materials. Herein, I also report high oxide-ion conductivity through interstitial oxygen (intrinsic oxygen vacancy) sites in Sillén oxychlorides, LaBi2-xTexO4+x/2Cl [8]. Oxide-ion conductivity of LaBi1.9Te0.1O4.05Cl is 20 mS cm−1 at 702 °C, and higher than best oxide-ion conductors as Bi2V0.9Cu0.1O5.35 below 201 °C. Despite of the presence of Bi and Te species, LaBi1.9Te0.1O4.05Cl shows extremely high chemical and electrical stability at 400 °C from oxygen partial pressure 10−25 to 0.2 atm and high chemical stability under CO2 flow, wet 5% H2 in N2 flow, and air with natural humidity. Neutron scattering length density analysis, DFT calculations, and ab initio molecular dynamics simulations indicate that the extremely high oxide-ion conduction is attributed to cooperative diffusion through interstitial oxygen sites (interstitialcy diffusion mechanism) in triple fluorite layers. The present findings demonstrate the ability of LaBi2-xTexO4+x/2Cl as superior oxide-ion conductors, which could open new horizons for oxide ion conductors.
Gielgud-I1
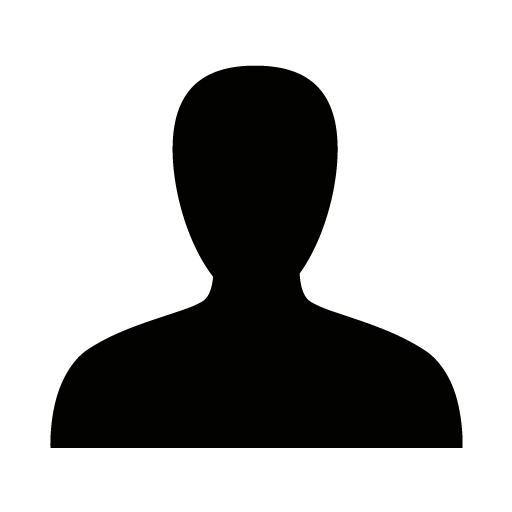
The surfaces of multicomponent perovskite oxides play a crucial role in many established and emerging technologies [1-5]. Yet, little is known about their atomic-scale details. The few systems that have been systematically investigated have shown a mindbogglingly rich structural variety on their surfaces [6-8].
Here, we focus on the two lowest-energy orientations of lanthanum strontium manganite (La0.8Sr0.2MnO3, LSMO). We grow (110)- [9] and (001)-oriented epitaxial thin films by pulsed-laser deposition (PLD) and characterize their surfaces using a variety of surface-science tools, most prominently scanning tunneling microscopy (STM).
(110)-oriented films exhibit a rich plethora of polarity-compensated reconstructions [10]. Which reconstruction appears on the surface and with which ratio is dictated by the surface composition and the oxygen chemical potential used to treat the sample [11].
On the other hand, (001)-oriented films display only two types of surface terminations over a broad range of parameters: one is rich in Mn, the other in La and Sr. The Mn-rich structure is particularly intriguing. In low-energy electron diffraction (LEED), it shows a 4-fold-symmetric pattern that cannot be explained by a set of two basis vectors as expected for a crystalline termination. A set of four, four-dimensional reciprocal-space vectors is needed instead – reminiscent of quasi-crystalline order. STM reveals an aperiodic real-space structure with a Fourier transform consistent with the LEED pattern. The reconstruction is well described as an incommensurately modulated structure [12].
Gielgud-K2
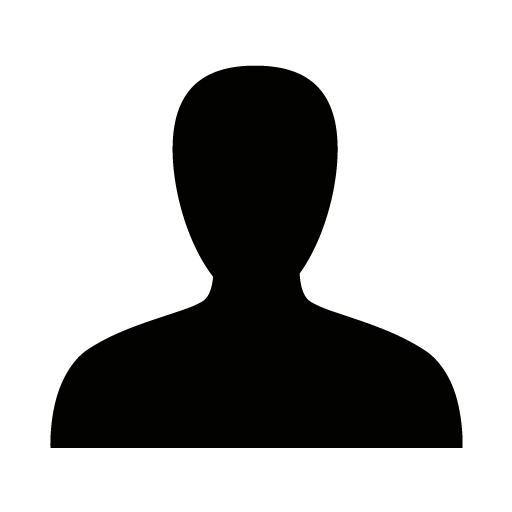
Modelling tools are well established in predicting defect and electronic properties of solid state ionic materials. Here we will highlight recent developments employing the concerted use of Periodic Boundary Conditions Quantum Mechanical, Hybrid, Quantum Mechanical / Molecular Mechanical (QM/MM) , and Static Lattice Interatomic Potential based methods, which are applied to widely studied oxide and nitride materials, including CeO2, ZnO, AlN and GaN. Our approach allows is to develop models of defect and electronic structures which are consistent with and rationalise experimental data. We gain new insights into the role of surface structure and composition in influencing defect and electronic properties), which we illustrate for the case of ceria. We predict charge carrier concentrations as a function of temperature and composition , where we report a detailed analysis for the case of ZnO. We are able to probe hole/electron - dopant interactions, where we discuss the factors controlling p-type conductivity in GaN,